Microfluidic technology: the next-generation drug discovery tool
Posted: 21 September 2015 | Kimberly Plevniak (Kansas State University), Mei He (Kansas State University)
Microfluidic miniaturisation, or the so-called ‘lab-on-a-chip’ concept, now encroaches on the fields of biology, medicine and pharmacology, and the nature of microfluidic technology (small volumes and high-throughput integration of fluid connections) means that it is outperforming conventional bench work. There has been an incredible need for microfluidic technology in the field of drug discovery. This article reviews current and future development of microfluidics in this field, particularly highlighting the frontiers in employing ‘organ-on-a-chip’ and exosome drug delivery systems for precise modelling of physiological microenvironments for drug development.
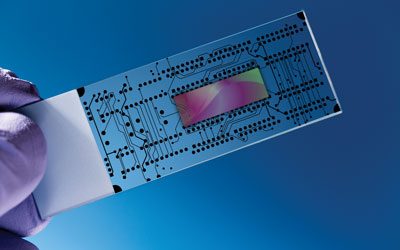
Before a drug becomes available for public use, it takes on average 15 years for it to become approved by regulatory agencies, from when it first starts its life at the drug discovery stage. In addition, a significant number of drug candidates fail during clinical trials, largely due to human toxicity that cannot accurately be addressed via current preclinical standards (e.g., in vivo tests). Substantial efforts have been made to eliminate unintended off-target effects during drug delivery, and so meet regulatory agency (e.g., the US Food and Drug Administration’s) standards. Consequently, developing an effective drug delivery system is key in bringing safe and effective drugs to market.
Microfluidic technology (the ‘lab-on-a-chip’ concept) has been a game changer in developing new drug delivery systems. Not only is this technology being used to create nanoparticles, but it also holds promise in developing microfluidic devices capable of replicating in vivo conditions in humans. This review will explore: the advantages of microfluidic systems for drug delivery; organ-on-a-chip technology; microfluidic exosome analysis for drug delivery blueprints; and cancer therapy. Particularly noteworthy is that the use of biomimetics alongside exosome study could be the solution that drug developers have been seeking over the last decade.
Microfluidics and drug delivery
Conventional drug delivery methods usually involve animal models, as well as two-dimensional (2D) monolayer cell cultures with intensive bench work. Microfluidic technology has shown prominent advantages over conventional drug delivery methods. These advantages relate to the small nature (e.g., micro or nano-scale) of microfluidic devices; the biologically relevant scales; the high degree of control geometry; and the precise control of microenvironment and fluidics2,3,4. Precise fluidic control plays a critical role when designing fluid delivery microenvironments; the design must take into account healthy and pathological conditions. Both the drug and delivery environments must be studied in order to fully understand the effects of certain drugs within physiological micro-environments2.
Offering great versatility, the design of microfluidic devices can vary depending upon the function as well as the material they are made out of, which can include polymers, glass, silicons, paper and metals. There are various microfabrication techniques with each of these materials. For instance, silicon and glass can be micro-fabricated using photolithography, vapour deposition, and chemical etching, while polydimethylsiloxane (PDMS) and poly (methyl methacrylate; PMMA) can be micro-fabricated using soft lithography techniques5. Such microfabrication techniques are readily available for designing microfluidic platforms employed for studying cell-based drug response and testing. Particularly, the gradient generating microfluidic platforms has been devised for testing drug response in time-evolving and steady-state.
Time-evolving gradient generators are composed of two reservoirs with high and low concentrations of one or more drugs of interest, then usually connected by a channel which house cells5. Steady-state gradient generators use continuous laminar flow to produce stable concentration gradients for investigating cell response to drugs over the long term5. Due to the flexibility in creating various gradient shapes upon time (e.g., tree-like gradient generator), this method is favourable for studying drug toxicity in longitudinal monitoring6.
Manipulating nanoparticles in microfluidic devices and understanding how nanoparticles interact in vivo is another important area to be considered whilst designing nanoparticles for drug delivery systems. Nanoparticles are transported through the bloodstream, distributed at the target site and taken up by cells, so all aspects of them need to be well understood to enable the design a successful drug delivery system1. Currently, the least toxic nanoparticle used for in vivo applications is lipid-based. There are two types of drug targeting systems in microfluidics that can be classified as either active or passive. Passive targeting aims to improve blood circulation time of the targeted drug delivery system; active targeting depends on the interactions between the drug and the intended target7.
Organ-on-a-chip
Through advances in 3D tissue engineering and microfluidics, a platform capable of reproducing in vivo microenvironments is now available. This organ-on-a-chip platform allows for cross-talking between cells, generating concentration gradients and fluid flow. Organs-on-chips are able to control complex interactions between different cell types such as cytokines, nutrients, growth factors, hormones, extracellular matrix and intracellular junctions8. One of the most innovative capabilities about the organ-on-a-chip is the real-time analysis of biological processes8. Thus, the organ-on-a-chip is currently employed for discovering new drugs and studying drug delivery process, though it is still in the early stages of development.
There have been several different organ-on-a-chip organs engineered to study variable drugs and conditions, such as lung-on-a-chip, liver-on-a-chip, tumour-on-a-chip, and cardiovascular chip. These engineered organs are normally created through the use of compartmentalised 3D microfluidic systems with cell culture on thin, porous elastomeric membranes8. The cultured cells can be stretched along channels and used to compartmentalise the device. Ideally, connecting all of the ‘organ-on-a-chip’ organ models would create the ultimate drug delivery test system for mimicking the human body.
Specific ‘on-chip’ examples
In vivo and in vitro organ-on-chips can help speed up the drug delivery process in a safe manner by precisely mimicking human physiological conditions. In terms of the lung-on-a-chip, the respiratory system is one of the most significant entry points of infection in the human body1 and such an entrance can serve as a good microenvironment for studying drug delivery processes; it has been shown that aerosol nanocomplexes are able to diffuse through the alveoli-capillary barrier efficiently1,9. However, nanoparticles behave differently when they are in static and flow conditions1,10,11. The conventional study of drug delivery in the lungs is through the use of 2D cell cultures, which is unable to reproduce physiological environments for drug testing. To create a truly ‘lung-like’ system with the proper fluid and solid mechanical forces1,12, a layer of alveolar epithelial and endothelial cells were seeded on opposite sides of a porous ECM-coated membrane1,10 in a microfluidic chip in one experiment. Using this organ-on-a-chip platform, the inflammatory and toxicity effects of silica nanoparticles were evaluated by using particle absorption through a vacuum, to simulate a breathing motion1,10.
A major concern when manufacturing a new drug is the potential for hepatotoxicity1. Developing in vitro models to study hepatoxicity and mimicking the functionality as well as pathophysiological architecture of the liver are absolutely necessary1. A hydrogel-based liver model has been established in a microfluidic chip for studying drug metabolism8. It is important to integrate liver-on-a-chip with other drug toxicity studies to assess the cytotoxicity of the drug delivery system1.
Recently, there has been an increased need for drugs that target cardiovascular disease. This is partially due to the fact that several cardiac drugs have been withdrawn from the market1,13, due to a lack of adequate screen models. However, mimicking the physiology of the heart in vitro is challenging1. A PDMS microfluidic device has nevertheless been developed with a hydrogel layer in controllable matrix stiffness, which is particularly important for cardiomycete cultures1,15. Such a platform can control the calcium dynamics of the cardiomyctes and monitor in real time1,14.
Microfluidic exosomes study
Exosomes, a unique population of nanoparticles of between 30-150nm in size, have risen as the most promising drug delivery carrier in recent years. It has been found that exosomes play an important role in intracellular communication and have strong ties to cancer cells. Exosomes that originate from tumour cells possess a different set of characteristics than exosomes from normal cells. Such unique characteristics can be used to increase the effectiveness of exosome-based drug delivery to cancer sites.
Due to the endogenous origin, exosomes have been observed as having a greater half-life over synthetic liposomal drug delivery carriers16. This advantage means exosomes could serve as possible blueprints for the development of future liposomal-based nanocarriers16. However, isolating exosomes for use can be challenging and time consuming (>10 hours). Bench top isolation methods include ultracentrifugation, sucrose gradient, filtration and immune-magnetic isolation. Among them, antibody-coated magnetic beads are the preferred technique, which produce a higher recovery and purity rate over using ultracentrifugation17. The cutting-edge microfluidic technology has shown unique advantages in isolating exosomes with much higher speed and efficiency, such as trapping exosomes with immune-affinity surfaces in microchannels, sieving using porous microstructures, and micro-scale control of immunomagnetic isolation18. Microfluidic platforms integrate exosome isolation with downstream molecular marker profiling, allowing on-line measurement of exosomal markers on surface and inside17. The specific circulating exosomes from the blood of patients can be directly detected and shows the high potential of exosomes for tracking and monitoring disease status and therapy.
Exosomes are also being used to target cancerous cells for drug delivery. In a recent clinical trial, exosomes were loaded up with MAG3 antigenic peptides and used as a vaccination therapy for stage III/IV melanoma patients16. Corroborating several other reports using exosome-based therapies, the exosomes exhibited excellent safety profiles within clinical settings16, and no toxicity was observed.
Meanwhile, microfabrication and microfluidic engineered exosomes have recently been developed for use as RNA and protein delivery vehicles for various therapeutic applications. Living cells in nature secrete only limited amounts of exosomes, and the collection process is complex and labour-intensive, making the exosomes not suitable in mass delivery of therapeutic elements to targeted cells. Generation of artificial nanovesicles and exosomes continuously in microfluidic devices is attractive, either through mechanical force breaking down cells or passing cells through hydrophilic microchannels19. This engineered nanovesicle is expected to be used in developing safer drug delivery systems.
Conclusion
There has been a huge need for microfluidics in the field of drug discovery, and this exciting technology has been found even to outperform conventional bench work. Employing ‘organ-on-a-chip’ and exosome drug delivery systems for precise modelling of physiological microenvironments is appealing for discovering future drugs. The development of a full ‘human-on-a-chip’ would defiantly revolutionise this process. More research into exosomes as blueprints for drug delivery also opens a new avenue for seeking safer, more effective and targeted drug delivery systems. Developing novel drug delivery systems will potentially lead to more drugs being available on the market and microfluidic technology will play an important role as the next-generation tool in facilitating future drug discovery.
References
- Bhise NS, Ribas J, Manoharan V, Zhang YS, Polini A, Massa S, Dokmeci MR, Khademhosseini A. Organ-on-a-chip platforms for studying drug delivery systems. J. Controlled Release. 2014, 190:82-93
- Bjoernmalm M, Yan Y, Caruso F. Engineering and evaluating drug delivery particles in microfluidic devices. J. Controlled Release. 2014, 190:139-149
- Squires TM, Quake SR. Microfluidics: Fluid physics at the nanoliter scale. Reviews of Modern Physics. 2005, 77:977-1026
- Qin D, Xia Y, Whitesides, GM. Soft lithography for micro- and nanoscale patterning. Nat. Protocol. 2010, 5: 491-502
- Zhang Y, Chan HF, Leong KW. Advanced materials and processing for drug delivery: The past and the future. Adv. Drug Deliv. Rev. 2013, 65:104-120
- Nam-Trung Nguyen, Shaegh SAM, Kashaninejad N, Dinh-Tuan Phan. Design, fabrication and characterization of drug delivery systems based on lab-on-a-chip technology. Adv. Drug Deliv. Rev. 2013, 65:1403-1419
- Khan IU, Serra CA, Anton N, Vandamme T. Microfluidics: A focus on improved cancer targeted drug delivery systems. J. Controlled Release. 2013, 172:1065-1074
- Esch EW, Bahinski A, Huh D. Organs-on-chips at the frontiers of drug discovery. Nat. Rev. Dr Discov. 2015, 14:248-260
- Zarogoulidis P, Karamanos NK, Porpodis K, Domvri K, Huang H, Hohenforst-Schmidt W, Goldberg EP, Zarogoulidis K. Vectors for Inhaled Gene Therapy in Lung Cancer. International Journal of Molecular Sciences. 2012, 13:17290-17291
- Huh D, Fujioka H, Tung Y, Futai N, Paine R, Grotberg JBIII, Takayama S. Acoustically detectable cellular-level lung injury induced by fluid mechanical stresses in microfluidic airway systems. Proc. Natl. Acad. Sci. U. S. A. 2007, 104:18886-18891
- Mahto SK, Yoon TH, Rhee SW. A new perspective on in vitro assessment method for evaluating quantum dot toxicity by using microfluidics technology. Biomicrofluidics. 2010, 4:034111
- Douville NJ, Zamankhan P, Tung Y, Li R, Vaughan BL, Tai C, White J, Christensen PJ, Grotberg JB, Takayama S. Combination of fluid and solid mechanical stresses contribute to cell death and detachment in a microfluidic alveolar model. Lab on a Chip. 2011, 11:609-619
- Nordt SP, Minns A, Tomaszewski C, Cantrell FL, Clark RF. Retrospective Review of Digoxin Exposures to a Poison Control System following Recall of Digitek (R) Tablets. American Journal of Cardiovascular Drugs. 2010, 10:261-263
- Martewicz S, Michielin F, Serena E, Zambon A, Mongillo M, Elvassore N. Reversible alteration of calcium dynamics in cardiomyocytes during acute hypoxia transient in a microfluidic platform. Integrative Biology. 2012, 4:153-164
- Annabi N, Selimovic S, Acevedo Cox, JP Ribas, J Bakooshli, MA Heintze, D Weiss, AS Cropek, D Khademhosseini A. Hydrogel-coated microfluidic channels for cardiomyocyte culture. Lab on a Chip. 2013, 13:3569-3577
- Johnsen KB, Gudbergsson JM, Skov MN, Pilgaard L, Moos, T Duroux M. A comprehensive overview of exosomes as drug delivery vehicles – Endogenous nanocarriers for targeted cancer therapy. Biochimica Et Biophysica Acta-Reviews on Cancer. 2014, 1846:75-87
- He M, Crow J, Roth M, Zeng Y, Godwin AK. Integrated immunoisolation and protein analysis of circulating exosomes using microfluidic technology. Lab on a chip. 2014, 14: 3773-3780
- Liga A, Vliegenthart ADB, Oosthuyzen W, Dear JW, Kersaudy-Kerhoas M. Exosome isolation: a microfluidic road-map. Lab on a chip. 2015: 15, 2388-2394
- Jo W, Jeong D, Kim J, Cho S, Jang SC, Han C, Kang JY, Gho YS, Park J. Microfluidic fabrication of cell-derived nanovesicles as endogenous RNA carriers. Lab on a chip. 2014,14:1261-1269
Biographies
Kimberly Plevniak has a BS degree in Molecular Biosciences from the University of Kansas. Currently she is a graduate student in the department of Biological and Agricultural Engineering at Kansas State University, pursuing her master degree. Her research interests include biomedical microfluidics and 3D printed diagnostic devices.
Dr Mei He is an assistant professor at the Kansas State University, directing a research group focused on defining sensitive elements in disease and personalised health conditions using biomedical microfluidic technology. Dr He received her PhD from the University of Alberta and completed her postdoctoral research from University of California, Berkeley. Dr He has published extensively in developing microfluidic technology and POC diagnosis in high impact journals, and has been granted four patents for technology transfer. Her research work has been recognised as the K-State Innovation by Johnson Cancer Research Center.
Related topics
Drug Delivery, Drug Discovery, Gene Therapy, Microfluidic Technology, Organ-on-a-Chip
Related organisations
Kansas State University
Related people
Kimberly Plevniak, Mei He