Building GPCR screening cascades for lead generation
Posted: 6 May 2014 | Erik Ryberg (AstraZeneca R&D), Linda Sundström (AstraZeneca R&D), Lovisa Frostne (AstraZeneca R&D), Niklas Larsson (AstraZeneca R&D)
Although this target class is already heavily investigated, there are still a significant number of opportunities for discovery of novel drugs targeting additional GPCRs. In this review, scientists from AstraZeneca R&D discuss the general build-up of GPCR screening cascades for discovery of chemical leads that can be further developed into new drugs…
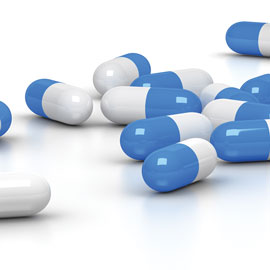
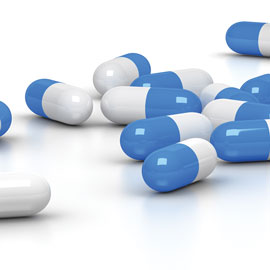
High quality drug discovery cascades are needed for the successful delivery of lead series that can be further optimised into drug candidates. In this mini-review, we discuss the design and development of screening cascades for lead generation on G protein-coupled receptors (GPCR) targets. The complex pharmacology of GPCRs and the distinct mechanisms required to treat different diseases underscore the need to select relevant cell models and tailor screening cascades to fit each individual receptor and indication.
GPCRs are among the most important target classes within the pharmaceutical industry, illustrated by the fact that a third of currently marketed drugs act on GPCRs1. Excluding olfactory and taste receptors, there are about 300 non-sensory GPCRs that represent potential drug targets. Of these, less than 50 are targets for drugs on the market2. Thus, although this target class is already heavily investigated, there are still a significant number of opportunities for discovery of novel drugs targeting additional GPCRs. In this review, we discuss the general buildup of GPCR screening cascades for discovery of chemical leads that can be further developed into new drugs. For general reviews on early drug discovery strategies, see Bleicher et al3 and Hughes et al4 for example.
Initial considerations for development of a screening cascade
During the last few decades, target-based approaches to drug discovery have dominated in the Pharma industry. The first step in such an approach is to establish a firm link between the target and the disease, where human patient based evidence is an essential part. In short, target validation includes genetic linkage analysis, confirmation that the target is expressed in tissues relevant for the disease and understanding of differences in expression pattern between healthy and diseased populations. In addition, investigation of the phenotypes of transgenic animals might demonstrate linkage between the target and the relevant mechanism. Next, both in vitro and in vivo pharmacological studies are performed to link the target to the disease. For unprecedented targets, such studies may be hampered by the lack of tool compounds. For GPCRs, it might be possible to get around this initial hurdle by using endogenous ligands. After selection of a target, a number of key questions need to be answered to design a screening cascade that enables identification of the desired type of molecules.
How druggable is the receptor?
A druggability assessment is generally performed before the start of a project to predict the chance of finding molecules that bind to and therapeutically regulate the function of a given GPCR. To simplify, a GPCR is predicted to be druggable if it is a member of a family where other members are known to be targeted by drugs, or if there is structural evidence suggesting cavities with physicochemical properties appropriate for binding of small molecules5. There are for example several drugs targeting biogenic amine receptors with small and polar endogenous ligands, representing a highly druggable GPCR subfamily. In contrast, discovery of molecules targeting GPCRs with peptide or protein ligands has been more challenging.
Which hit finding strategy should be used?
Availability of compounds active on the target and druggability assessment together with receptor structure information (if available) and homology models guides which hit finding strategy to apply. During the last 20 years, high-throughput screening (HTS) has been the dominant strategy to identify novel chemical starting points hitting GPCRs. Today, HTS in the pharmaceutical industry means screening compound libraries of up to a few million compounds. Sharing of libraries with different origin between companies and institutions also occurs to further increase the likelihood to find relevant hits6. Alternatives to the HTS approach are strategies like focused screens using compounds predicted to be active on the target. Such compounds can be selected based on similarity to endogenous ligands, patent and literature compounds or virtual screens using three dimensional models of the target. As more GPCR structures are being published, the use of structure based approaches are starting to increase, in most cases employing site-directed mutagenesis to stabilise receptors and make them suitable for crystallisation7,8.
Which molecular mechanism of action is required to treat the disease?
As illustrated in Figure 1B, GPCR ligands can have different modalities and trigger responses with different levels of efficacy. It is essential to understand the modality and level of efficacy that is required to achieve a desired therapeutic effect. However, such understanding is also vital for development of relevant cell reagents and assays when building a GPCR screening cascade.
Historically, ligands were either classified as agonists that activate receptors, or antagonists that block the response caused by an agonist. We now know that many receptors have an intrinsic constitutive activity and that ligands can induce a range of efficacies, from full agonism via neutral antagonism to full inverse agonism. In addition, potency and efficacy of ligands can be positively and negatively modulated via allosteric interactions9. An assay measuring the activity of allosteric modulators is one specific challenge. The ability to fine-tune assay / screening platforms to quantify allosteric effects is not routine. Moreover, it is challenging to fit experimental data to mass-action parameters and drive structure-activity relationships (SAR) that can be translated to a meaningful therapeutic outcome10,11.
Which signalling pathways are important for treatment of the disease?
The historical concept has been that a given receptor acts through only one Gα subtype-linked effector system. Today, it is well accepted that ligands can activate several signalling pathways downstream of a specific receptor (Figure 1A). Moreover, different ligands acting on the same receptor can have different abilities to activate distinct pathways, a phenomenon called agonist-trafficking or biased signalling12 (Figure 1C).
There are drugs on the market where biased signalling has been demonstrated to play a role in the clinic13. One example is the non-selective β-blocker carvedilol; a nonsubtype-selective β-Adrenoceptor ligand used for treatment of heart failure. Carvedilol is an inverse agonist with respect to Gαs-mediated cAMP production and at the same time an agonist regarding β-arrestin mediated signalling downstream of β1 and β2-AR, with beneficial cardioprotective effects14. This example of ligand-selective signalling underscores the complexity of GPCR signalling that needs to be understood to effectively design a GPCR screening cascade that will deliver relevant lead series. However, one key challenge for build-up of screening cascades often exists: how to establish, at an early stage, the downstream pathways relevant to hit to achieve beneficial effects in the clinic.
Building the screening cascade
The aim with a screening cascade in the lead generation phase is to identify and select the lead series with most potential to be optimised into drugs. The cascade starts with a primary screen that is used to identify compounds acting on the target (Figure 2). Selecting the right primary screen is crucial since its ability to detect compound activity at the target will depend on the chosen assay and compounds not picked up will be lost. If the hit finding strategy builds on HTS, the primary assay needs to be robust enough and have sufficient throughput to cover up to a few million compounds tested at one concentration. High throughput technologies commonly applied include assays that measure direct receptor-ligand binding or changes in GPCR downstream signalling events such as cAMP or inositol phosphate production, Ca2+-flux, β-arrestin recruitment and ERK phosphorylation (Figure 1A).
There are drawbacks and benefits with all technologies. Binding assays have limitations outside orthosteric site binders and give no information regarding pathways or efficacy. On the other hand, binding assays can provide kinetic data, such as association and dissociation rates, not easily obtained with functional assays. Many assays measuring GPCR downstream signalling events are commercially available, simple to apply and robust. However, individual technologies in most cases measure signalling via one single pathway. If a single pathway assay is applied for primary screening, it is crucial to establish that this pathway is relevant for treatment of the disease. Available ligands should therefore be tested in disease relevant biological effect assays already during development of the cascade to verify that the primary assay is capable of identifying compounds with the desired pathway profile/bias and mechanism of action.
β-arrestin recruitment assays are able to capture activation of most GPCRs irrespective of G-protein coupling preference and can therefore be applied as a general platform for GPCR hit finding15. With this approach, one needs to take into account that biased compounds with effect mainly on the β-arrestin pathway are found whereas other relevant actives may be missed, similar to single pathway assays. More recently, cell based label-free assays have been developed that capture the integrated cell response and are less biased towards the specific downstream signalling pathways. Such technologies either employ optical (resonant waveguide grating; RWG) or impedance-based biosensors (cell impedance spectroscopy; CIS)16,17. Although covering multiple pathways, the drawback with label-free approaches is the black box nature of the readout and requirement for subsequent dissection of pathways and mechanisms.
All technologies mentioned above can be applied for primary screening, and the choice of technology will depend on the answers to the initial key questions asked above.
Subsequent to the primary screen, a counterscreen using an artefact assay is often applied to identify and remove compounds that are false actives in the primary assay due to interference with the assay technology or off-target activity. An artefact assay mirrors the primary assay by using the same technology and cell type except for the target receptor. Especially for hit finding strategies that involve screening of large libraries, false actives are a general concern. Counterscreening may therefore be complemented with secondary screening using an orthogonal assay employing an independent technology to verify compound activity at the target receptor.
It is key to establish that compound activity identified in primary and orthogonal assays can be translated into a meaningful biological effect, measured in native(like) cells relevant for the disease. Interpretation of potency and efficacy data obtained using cellular assays must be done with caution since the cellular context will affect the observed pharmacology. For example, GPCR assays are commonly run using transfected HEK293 or CHO cell lines overexpressing the receptor of interest. Such systems often contain a ‘receptor reserve’, meaning that the maximal functional response is already reached when only a fraction of the receptors are occupied. In practice, the consequence is that potency and/or efficacy of agonists are overestimated when overexpressing cell lines are used. Our experience is that successful delivery of lead series is closely related to availability of a disease relevant biological effect assay early in the cascade.
Pathway profiling assays are included to characterise ligand bias for receptors coupling via multiple pathways since biased compounds can be of therapeutic advantage13. Selectivity assays are used to establish selectivity versus other targets to avoid adverse effects. Selection of key selectivity targets is based on homology to the receptor and/or ligand similarities. Assays measuring compound activity at species orthologs of the target receptor are employed to address translation to species prior to testing of compounds in pharmacokinetic and pharmacodynamic in vivo models, often rat and mouse. Furthermore, if the biological effect assay can’t be performed in cells of human origin, species assays are moved upwards in the cascade compared to the scheme in Figure 2, and applied to link in vitro pharmacology between recombinant and native(like) cell models.
Besides target-related assays described in Figure 2, in vitro DMPK parameters are also critical for selection of lead series to be further optimised. Finally, series representatives are characterised with respect to molecular mechanism of action (MMoA), e.g. kinetics / reversibility / competitiveness, typically using a binding assay.
Although an iterative process, we have illustrated lead generation in a simplified linear way in Figure 2. Initial cycles employ only primary and artefact assays at the top of the cascade, to expand hit series using internally and commercially available nearest neighbours and combinatorial libraries. As series are identified and prioritised for chemical synthesis and further SAR development, the full cascade of assays is used in an iterative way to support a ‘design-make-test-analysis’ (DMTA) cycle for compound evolution.
Concluding remarks and future perspectives
GPCRs remain one of the major target classes for the pharmaceutical industry. To increase efficiency and reduce cost of drug discovery, it is tempting for companies to apply streamlined generic screening platforms for GPCRs. However, each receptor and drug hunting project has its individual requirements. This, together with the complexity of GPCR pharmacology (e.g. biased signalling) underscores the need to tailor hit finding strategies and screening cascades to fit individual receptors.
Finally, there are exciting opportunities to enhance the design of screening cascades to successfully deliver relevant lead series as new disease cell models are being developed. Recent advances in the induced pluripotent stem (iPS) cell18 and precise genome modification19 fields open up possibilities to generate tissue specific cells with endogenous expression levels of receptors. Such cells have the potential to provide relevant measures of efficacy and potency early in cascades or even be used for primary screening. Precise genome modification also makes it possible to generate isogenic control knock-out or knock-in cells to be used for counterscreening. We believe that the combination of easier access to biological effect cell assays and early confirmation of target specific chemical series will increase the chance to successfully deliver relevant lead series and reduce costly late attrition.
References
- Imming P, Sinning C, Meyer A. Drugs, their targets and the nature and number of drug targets. Nat.Rev.Drug Discov. 2006 Oct;5(10):821-834.
- Lagerstrom MC, Schioth HB. Structural diversity of G protein-coupled receptors and significance for drug discovery. Nat.Rev.Drug Discov. 2008 Apr;7(4):339-357.
- Bleicher KH, Bohm HJ, Muller K, Alanine AI. Hit and lead generation: beyond high-throughput screening. Nat.Rev.Drug Discov. 2003 May;2(5):369-378.
- Hughes JP, Rees S, Kalindjian SB, Philpott KL. Principles of early drug discovery. Br.J.Pharmacol. 2011 Mar;162(6):1239-1249
- Hajduk PJ, Huth JR, Tse C. Predicting protein druggability. Drug Discov.Today 2005 Dec;10(23-24):1675-1682
- Kogej T, Blomberg N, Greasley PJ, Mundt S, Vainio MJ, Schamberger J, et al. Big pharma screening collections: more of the same or unique libraries? The AstraZeneca-Bayer Pharma AG case. Drug Discov.Today 2013 Oct;18(19-20):1014-1024
- Salon JA, Lodowski DT, Palczewski K. The significance of G protein-coupled receptor crystallography for drug discovery. Pharmacol.Rev. 2011 Dec;63(4):901-937
- – Andrews SP, – Tehan B. – Stabilised G protein-coupled receptors in structure-based drug design: a case study with adenosine A2A receptor. – Med. Chem. Commun. 2013 Jan; (4):52-67
- Kenakin T. Principles: receptor theory in pharmacology. Trends Pharmacol.Sci. 2004 Apr;25(4):186-192
- Keov P, Sexton PM, Christopoulos A. Allosteric modulation of G protein-coupled receptors: a pharmacological perspective. Neuropharmacology 2011 Jan;60(1):24-35
- Wootten D, Christopoulos A, Sexton PM. Emerging paradigms in GPCR allostery: implications for drug discovery. Nat.Rev.Drug Discov. 2013 Aug;12(8):630-644
- Violin JD, Lefkowitz RJ. Beta-arrestin-biased ligands at seven-transmembrane receptors. Trends Pharmacol.Sci. 2007 Aug;28(8):416-422
- Whalen EJ, Rajagopal S, Lefkowitz RJ. Therapeutic potential of beta-arrestin- and G protein-biased agonists. Trends Mol.Med. 2011 Mar;17(3):126-139
- Wisler JW, DeWire SM, Whalen EJ, Violin JD, Drake MT, Ahn S, et al. A unique mechanism of beta-blocker action: carvedilol stimulates beta-arrestin signaling. Proc.Natl.Acad.Sci.U.S.A. 2007 Oct 16;104(42):16657-16662
- Southern C, Cook JM, Neetoo-Isseljee Z, Taylor DL, Kettleborough CA, Merritt A, et al. Screening beta-arrestin recruitment for the identification of natural ligands for orphan G-protein-coupled receptors. J.Biomol.Screen. 2013 Jun;18(5):599-609
- Cooper MA, Halai R. What is label-free screening and why use it in drug discovery? European Pharmaceutical Review 2012;17(6):51-53
- Larsson N, Sundström L, Ryberg E, Frostne L. GPCRs: Cell based label-free assays in GPCR drug discovery. European Pharmaceutical Review 2013;18(4):13-16
- Cherry AB, Daley GQ. Reprogrammed cells for disease modeling and regenerative medicine. Annu.Rev.Med. 2013;64:277-290
- Wei C, Liu J, Yu Z, Zhang B, Gao G, Jiao R. TALEN or Cas9 – Rapid, Efficient and Specific Choices for Genome Modifications. Journal of Genetics and Genomics 2013 6/20;40(6):281-289
Biographies
Niklas Larsson obtained his PhD 1999 in Cell and Molecular Biology at Umeå University, Sweden. Since 2000, he has been working with early drug discovery projects at AstraZeneca (Mölndal, Sweden) in different positions such as Project Leader, Team Leader and currently as Associate Principle Scientist within the Discovery Sciences function. His scientific focus is drug discovery and molecular pharmacology of GPCRs.
Erik Ryberg obtained his PhD in Biomedical Science at the University of Aberdeen, Scotland in 2009. He has been working with GPCR related drug discovery at AstraZeneca for over 10 years in different positions. His current position is Associate Principal Scientist within the Cardiovascular and Metabolic Diseases function, with primary focus on molecular pharmacology of GPCRs in different phases of drug discovery.
Linda Sundström obtained her MSc in Chemical Engineering at Lund Institute of Technology, Sweden. She completed her thesis work at Institute of Biotechnology, University of Cambridge, UK. For more than 12 years, she has been working with GPCR targets in early drug discovery at AstraZeneca, Sweden. She currently holds the position of Team Leader for a screening team within Discovery Sciences.
Lovisa Frostne joined AstraZeneca (Mölndal, Sweden) in 2003 after obtaining her MSc in Molecular Biology at the University of Gothenburg, Sweden. She completed her thesis work at the Institute for Molecular Bioscience at the University of Queensland, Australia. During her 10 years in AstraZeneca her work has focused around GPCR pharmacology in early drug discovery. She is currently a Team Leader in the Bioscience department within the Respiratory, Inflammation and Autoimmune iMed.
Related topics
Drug Discovery, Drug Targets, GPCRs, Lead Generation, Screening
Related organisations
AstraZeneca
Related people
Erik Ryberg, Linda Sundstrom, Lovisa Frostne, Niklas larsson