Protein Expression: The growing potential of plant-made monoclonal antibodies
Posted: 3 December 2015 | Huafang Lai (Arizona State University), Qiang “Shawn” Chen (Arizona State University) | No comments yet
The success story of Mapp Pharmaceuticals’ experimental drug, ZMapp, during last year’s Ebola outbreak highlights the potential of plant-made monoclonal antibodies (mAbs) as life-saving treatments.
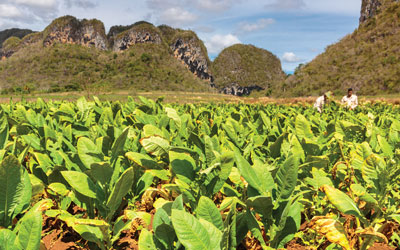
Current plant expression systems offer features beyond the traditional advantages of eukaryotic protein modification, such as low cost, high scalability and increased safety. The development of novel transient expression vectors has allowed mAbs to be produced at unprecedented speed to mitigate potential pandemics. Glycoengineering allows plants to make mAbs with unique mammalian glycoforms with differential binding to various Fc receptors (FcγRs), providing new utility to make biobetters with superior potency or safety. Along with ZMapp, several human clinical trials have been conducted. Ultimately, obtaining regulatory approval is key to fulfilling the vast commercial potential of this technology.
Since the commercialisation of the first therapeutic mAb in 1986, they have represented the largest and fastest-growing class of biopharmaceutical products. The development of mAbs has revolutionised the pharmaceutical industry, created a multibillion dollar market, and provided vast opportunities to treat a wide range of diseases from autoimmune disorders to cancer to viral or bacterial infections. MAbs produced in mammalian cell culture have achieved remarkable pharmaceutical and financial success leading to several blockbuster therapeutic products. However, they are prohibitively expensive, making them unaffordable for the majority (>75% population) of countries around the globe1. MAbs produced in mammalian cell cultures require capital-intensive facilities, fermenters, expensive downstream processing, cold storage and transportation, and sterile delivery methods2-4. The high cost of this system has encouraged the development of alternative production systems including yeast, insect cells and plants. While there is much progress being made with these systems, the general public has very little knowledge about them and their utility in producing mAb drugs for human use.
ZMapp’s full potential revealed in Liberia
Early in 2014, a new strain of the Zaire species of Ebola virus, one of the world’s most deadly pathogens, emerged in Guinea, West Africa, and rapidly spread to Liberia, Sierra Leone and Nigeria. It was the largest Ebola outbreak in history, infecting 28,512 people with 11,313 fatalities to date5. Out of this total devastation, a dramatic chapter came from the survival of two American health aid workers, Dr. Kent Brantly and Nancy Writebol, who contracted Ebola at the Liberian hospital. Brantly’s condition had deteriorated to the point where he thought that he was dying and called his wife to say goodbye. At this critical moment, he was given an intravenous dose of a little-known, experimental drug called ZMapp. What happened next was described as ‘miraculous’ by his doctors: Brantly’s breathing became easier, and he was able to take a shower without any assistance by the next morning. Similarly, Nancy Writebol’s condition also dramatically improved after receiving two doses of ZMapp6.
The story of Brantly and Writebol became a media sensation and people became intrigued about the nature of ZMapp. The drug is a cocktail of three chimeric mAbs made in Nicotiana benthamiana plants (Figure 1). The fact that it is produced in plants is both fascinating and surprising for most people – how can a drug that might save people’s lives come from tobacco, a plant has done so much harm to people’s health?
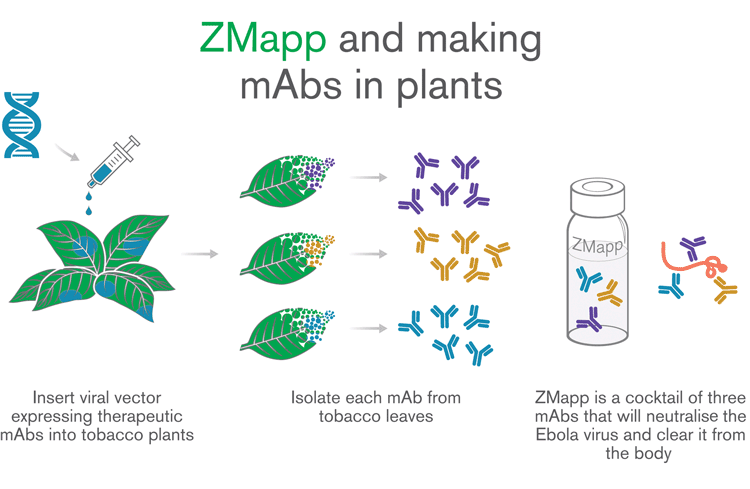
Figure 1: Production of ZMapp in tobacco plants
But plants, especially tobacco and its related Nicotiana plants, have been used to produce mAbs for decades, with the first mAb made in tobacco in 19897. Despite the complexity of mAb production requiring the assembly of four polypeptides into a heterotetramer and complex glycosylation, a variety of mAbs and their derivatives have been produced in many plant species, such as secretory IgAs, tetravalent mAbs, immune-complex, single-domain fragments, single-chain variable fragments (scFv), and diabodies. An increasing number and diversity of MAbs are being produced every year and several of them have now reached human clinical trials4.
However, until now, these efforts were largely unknown beyond the close-knit field of plant-made pharmaceuticals (PMPs). The intense media interest in ZMapp has given the public unprecedented exposure to the fact that PMPs can be life-saving drugs, and an international stage that we, who have worked in the PMP field for many years, could never have anticipated. We are repeatedly asked by the media and general public – why plants? Why tobacco and how can this work?
Why use plants?
The traditional advantages of utilising plants to produce mAbs are their demonstrated low cost, high scalability and increased safety2,8, while a major advantage of plant-production systems is their low-cost upstream protein expression9. In contrast to mammalian cell culture systems, which require capital-prohibitive bioreactors and expensive culture media, plant mAb production can be easily scaled in inexpensive green houses. Tobacco plants can be grown in soil or hydroponically in simple mineral solutions to produce a large amount of biomass and millions of seeds per plant for a facile scale-up of production, making high levels of mAbs efficiently and sustainably4.
Plants rarely carry human or animal pathogens, reducing the risk of contamination. In addition, plants’ cellular protein machinery can easily assembly multiple subunits required for the production of mAbs and efficiently perform critical post-translational modifications4.
New innovations in viral vector development provide additional benefits for plants to make mAbs. For example, the development of the ‘deconstructed’ viral vector system has successfully addressed the challenges of sufficient protein expression levels, consistency and speed of mAb production in plants10-12.
In contrast to using stable transgenic plants, transient expression with deconstructed viral vectors avoids the months-long process of generating and selecting transgenic plants. It can produce up to 5mg mAb per gram of fresh leaf weight (LFW) within two weeks due to the robust transcriptional and translational activities of the plant virus components in the vector11,13. These deconstructed viral vectors are non-competitive, allowing mAbs and other proteins with more than one hetero-subunit to be expressed and assembled in one individual cell11,13,14.
Agrobacterium tumefaciens, a bacterium that naturally transfers a portion of its plasmid into plant cells, is used to deliver the deconstructed viral vectors to plant cells15-17. Thus, the deconstructed viral vector system provides the flexibility of nuclear gene expression with the speed and high-yield of viral vectors. The rapid and high level mAb production capability of the transient expression systems make them the system of choice for obtaining the milligram and gram levels of mAbs needed for preclinical studies. These vectors have also been adapted for scale-up manufacturing of mAbs in stable transgenic plants, while retaining consistent and high mAbs expression level and transgene stability2. Therefore, a rapid evaluation of mAb candidates and transition to a large-scale commercial production can be accomplished by the combinatorial use of both transient and stable plant expression based on deconstructed viral vectors.
N-glycosylation in plants is similar to human cells yet has minor differences. These differences were once a cause for concern, since they may produce plant glycan-specific antibodies that could reduce therapeutic efficacy or cause adverse effects. But glycoengineering of host plants via knocking out/down plant-specific glycan genes or/and inserting mammalian glycosylation genes has overcome this challenge18,19. Extensive studies have shown that mAbs produced in the glycoengineered plants do not contain any plant-specific xylose or 1,3-frucose, but have authentic mammalian N-glycans18,19. Astonishingly, these mAbs also have a remarkably high degree of glycan uniformity that cannot be produced by mammalian cells or achieved by in vitro treatments20-23. A portfolio of plant lines has been developed, with each producing mAbs with a unique mammalian N-glycoform and providing a potential system for producing mAbs with defined, uniform carbohydrate moieties based on their functional needs.
Leading candidates for plant-derived mAbs
The early work of plant-made mAbs against Ebola that contributed to the eventual development of ZMapp started at Arizona State University (ASU). ASU had received several large grants from US federal agencies to develop mAbs or vaccines against Ebola virus24. Due to the deadly nature of Ebola virus, the use of plant-based mAb therapeutics and vaccines meets a critical public health challenge and builds an important biodefense stockpile. Three mAbs: 6D8, 13C6 and 12F6, were initially expressed in N. benthamiana plants. Our characterisation showed that fully assembled mAbs can be detected just two days following infiltration (DPI) of viral vectors and reached the peak accumulation at 4 DPI up to 0.5mg/g LFW14 (Figure 2).
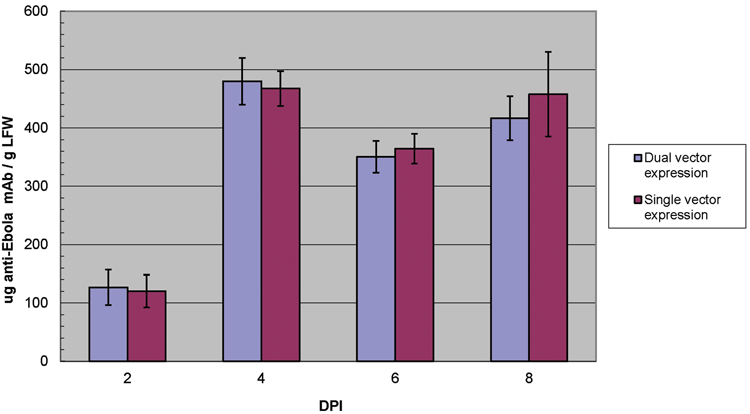
Figure 2: Expression of anti-Ebola mAb in N. benthamiana plants with geminiviral dual- or single-vector system. Levels of mAb accumulation in leaves days post vector infiltration (DPI) was measured by ELISA
Our results also indicate that these mAbs can be produced in lettuce with a comparable accumulation level and rapidity as in N. benthamiana25. These mAbs can be effectively purified to greater than 95% purity by simple chromatographic steps14,25,26. The successful use of commercially-produced lettuce demonstrated a viable option of supplying an almost unlimited amount of cost-effective material for large-scale production of anti-Ebola mAb therapeutics. Both tobacco and lettuce-produced mAbs retain their specific affinity for Ebola GP protein, suggesting they are functionally active14,25 (Figure 3).
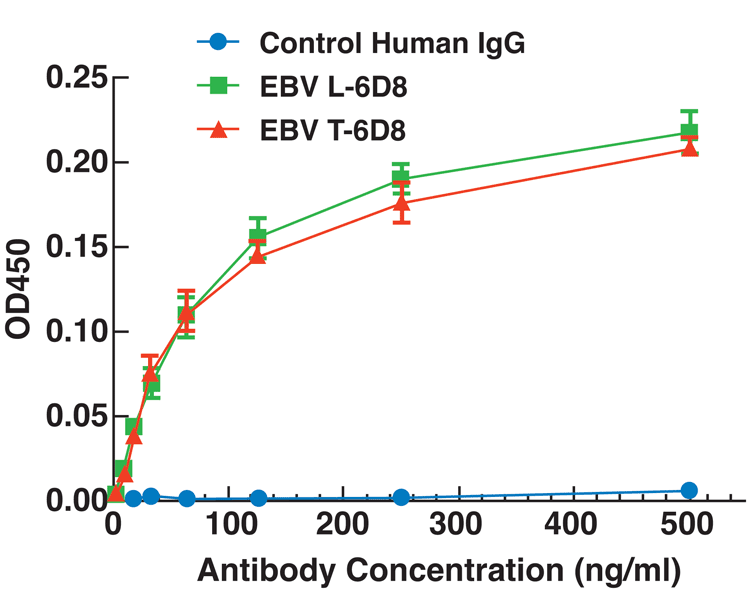
Figure 3: Lettuce (L) or tobacco (T)-produced 6D8 mAb shows specific binding to irradiated Ebola virus
Our colleagues at Mapp Biopharmaceutical demonstrated the in vivo efficacy of these mAbs and in concert with our efforts, demonstrated that a vaccine candidate based on these mAbs also protected mice from a lethal challenge of Ebola27-29. The efficacy of a cocktail of the three plant-made mAbs (6D8, 13C6 and 12F6), called MB-003, was subsequently established in nonhuman primates30. Remarkably, the mouse and macaque experiments also revealed that plant-derived individual anti-Ebola mAb and MB-003 have a superior potency to their mammalian-produced counterparts, most likely due to the fact that they carry the homogenous GnGn mammalian glycans, in contrast to the heterogeneous glycan populations of the mammalian cell-derived mAbs27,30. This paved the way for combining one mAb from MB-003 with two other mAbs to formulate ZMapp and its experimental use in Brantly, Writebol and five other human patients during the Ebola outbreak. A subsequent report proved that ZMapp is able to rescue 100% of rhesus macaques even when given five days following lethal Ebola challenge31. A clinical trial with the drug is now being conducted in Liberia by the US Institutes of Health (NIH) to examine its safety and efficacy.
The rapid nature of plant transient expression can also facilitate new treatments for cancer. This includes the development of a treatment for non-Hodgkin’s lymphoma (NHL), a group of blood cancers that have a significant impact on global health. The extremely variable nature of NHL calls for the development of a patient-specific immunotherapeutic approach through vaccination with the patient’s own idiotype immunoglobulin (Ig). This requires a production platform that offers the flexibility to rapidly produce tailor-made, patient- and tumour-specific antigens at low cost. These requirements present a difficult obstacle for mammalian cell cultures, but can be readily met by plant transient expression.
In fact, idiotype-specific scFvs of the Ig from the 38C13 mouse B cell lymphoma can be expressed in plants within two weeks of vector inoculation, which protect 90% of mice against a lethal challenge of the syngeneic 38C13 tumour32. Subsequently, 44 human tumour-specific scFvs were rapidly produced, and were shown to recognise the Ig on human tumour cells and induce idiotype responses with minimal cross reactivity to irrelevant Igs33. The safety and immunogenicity of these scFv-based personalised vaccines were tested in a phase I clinical trial34. The results indicated that all individualised scFv vaccines were well-tolerated by all patients34. Greater than 70% of the patients developed humoral or cellular immune responses34. Thirteen of 16 patients remained alive with a median follow-up of 78 months. These findings suggest that plant-produced idiotype vaccines are feasible to produce, safe to administer, and provide a viable option for idiotype-specific immune therapy in follicular lymphoma patients.
The availability of a collection of plant lines that produce mAbs with uniform and distinct mammalian glycoforms also offers opportunities to address the safety issue of mAb therapeutics against flaviviruses, which are prone to the development of so-called antibody-dependent enhancement of infection (ADE). ADE may render anti-flavivirus mAb-treated subjects more susceptible to infection. ADE occurs because sub-neutralising concentrations of antibodies (including therapeutic mAbs) form complexes with the infecting flavivirus that bind to FcγR-bearing cells, resulting in increased virus uptake and infection35. To address this major impediment, our laboratory has developed a plant-derived mAb (E16) against West Nile virus (WNV) with various unique N-glycoforms20-23. Our results indicate that a single dose of plant-made E16 protected mice from lethal infection of WNV, even given four days post infection23. Furthermore, in contrast to mammalian cell-made E16, all plant E16 glycovariants lost ADE activity on CD32A-expressing K562 cells (Figure 4). Thus, mammalian forms of glycans carried by plant-produced E16 mAb do not induce the development of ADE, consistent with their in vitro binding pattern as shown by SPR20. These results demonstrate the potential of plant-made mAbs and their variants to be used as therapeutics against ADE-prone viruses.
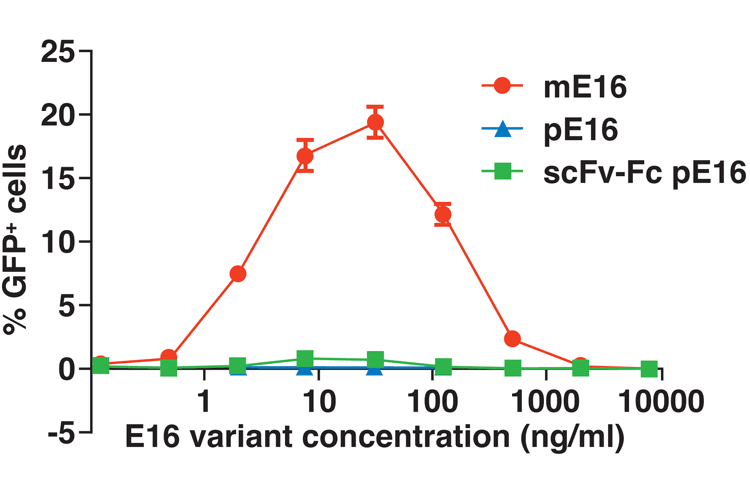
Figure 4: Plant-produced anti-WNV E16 mAb glycovariants (pE16 and scFv-Fc pE16) have no or greatly reduced risk of ADE compared with mammalian cell-produced E16 mAb (mE16)
Conclusions
Plant expression systems not only offer the traditional advantages of proper eukaryotic protein modification, low cost, high scalability and increased safety, but they also allow the production of mAbs at unprecedented speed to control potential pandemics or with specific glycoforms for superior potency or safety. These advantages offer plants as a superior alternative production system for mAb production. Plants can be used to produce mAb biosimilars because of their large capacity to rapidly generate mAb at low cost, but can also be explored to produce safer and more effective mAb biobetters due to the flexibility of producing mAbs with specific and unique mammalian glycoforms that preferentially bind to various FcγRs. The approval of the first PMP by the US FDA for treating Gaucher’s disease has established the regulatory pathway and warmed up the interests of large pharmaceutical companies in the PMPs, fueling new hopes for the field36,37. The successful story of ZMapp has sparked new interests and promoted several large government investments to expand the capacity of producing mAbs from plants under cGMP regulations38-40. We speculate that we should see the regulatory approval of the first few plant-made mAb-based therapeutics or vaccines within the next decade.
Acknowledgements
The authors thank J. Caspermeyer for the critical reading of the manuscript.
References
- G Walsh. Biopharmaceutical benchmarks 2014. Nat Biotechnol. 2014; vol. 32, no. 10, pp. 992-1000
- Q Chen. Expression and manufacture of pharmaceutical proteins in genetically engineered horticultural plants. Transgenic Horticultural Crops: Challenges and Opportunities – Essays by Experts, B Mou and R Scorza, Ed., pp. 83-124, Taylor & Francis Boca Raton, 2011
- Q Chen. Turning a new leaf. European Biopharmaceutical Review vol. 2, no. 56, pp. 64-68, 2011
- Q Chen and H Lai. Plant-derived monoclonal antibodies as human biologics for infectious disease and cancer. Plant-derived Pharmaceuticals: Principles and Applications for Developing Countries, KL Hefferon, Ed., pp. 42-75, CABI, Cryodon, UK, 2014
- Ebola situation report, October 21, 2015, World Health Organization 2015, pp. 1-16
- E Check Hayden & S Reardon. Should experimental drugs be used in the Ebola outbreak? Nature (London). 2014; vol. http://dx.doi.org/10.1038/nature.2014.15698
- A Hiatt, R Cafferkey & K Bowdish. Production of antibodies in transgenic plants. Nature. 1989, vol. 342, no. 6245, pp. 76-78
- Q Chen, C Zhang and L Santi. Plant-Made Biologics. BioMed Research International, 2014; vol. 2014, no. DOI: 10.1155/2014/418064, pp. 3
- D Tuse, T Tu and K McDonald. Manufacturing Economics of Plant-Made Biologics: Case Studies in Therapeutic and Industrial Enzymes. BioMed Research International. 2014; vol. 2014, no. DOI: 10.1155/2014/256135, pp. 10
- Z Huang, Q Chen, B Hjelm, C Arntzen and H Mason. A DNA replicon system for rapid high-level production of virus-like particles in plants. Biotechnology and Bioengineering. 2009; vol. 103, no. 4, pp. 706-714
- Q Chen, J He, W Phoolcharoen and HS Mason. Geminiviral vectors based on bean yellow dwarf virus for production of vaccine antigens and monoclonal antibodies in plants. Human Vaccines, 2011; vol. 7, no. 3, pp. 331-338
- C Lico, Q Chen and L Santi. Viral vectors for production of recombinant proteins in plants. Journal of cellular physiology. 2008; vol. 216, no. 2, pp. 366-377
- A Giritch, S Marillonnet, C Engler, G van Eldik, J Botterman, V Klimyuk & Y Gleba. Rapid high-yield expression of full-size IgG antibodies in plants coinfected with noncompeting viral vectors. Proceedings of the National Academy of Sciences of the United States of America. 2006; vol. 103, no. 40, pp. 14701-14706
- Z Huang, W Phoolcharoen, H Lai, K Piensook, G Cardineau, L Zeitlin, K Whaley, CJ Arntzen, H Mason and Q Chen. High-level rapid production of full-size monoclonal antibodies in plants by a single-vector DNA replicon system. Biotechnology and bioengineering, 2010; vol. 106, no. 1, pp. 9-17
- K Leuzinger, M Dent, J Hurtado, J Stahnke, H Lai, X Zhou and Q Chen. Efficient Agroinfiltration of Plants for High-level Transient Expression of Recombinant Proteins. Journal of Visualized Experiments. 2013; no. 77, pp. doi:10.3791/50521
- Q Chen and H Lai. Gene delivery into plant cells for recombinant protein production. BioMed Research International, 2014; vol. 2014, no. DOI: 10.1155/2014/932161, pp. 10
- Q Chen, H Lai, J Hurtado, J Stahnke, K Leuzinger and M Dent. Agroinfiltration as an Effective and Scalable Strategy of Gene Delivery for Production of Pharmaceutical Proteins. Advanced Technolgy in Biology and Medicine. 2013; vol. 1, no. 1, pp. 103-112
- M Schahs, R Strasser, J Stadlmann, R Kunert, T Rademacher and H Steinkellner. Production of a monoclonal antibody in plants with a humanized N-glycosylation pattern. Plant Biotechnol J. 2007; vol. 5, no. 5, pp. 657-663
- R Strasser, J Stadlmann, M Schahs, G Stiegler, H Quendler, L Mach, J Glossl, K Weterings, M Pabst and H Steinkellner. Generation of glyco-engineered Nicotiana benthamiana for the production of monoclonal antibodies with a homogeneous human-like N-glycan structure. Plant Biotechnology Journal, 2008; vol. 6, no. 4, pp. 392-402
- J He, H Lai, M Engle, S Gorlatov, C Gruber, H Steinkellner, MS Diamond and Q Chen. Generation and Analysis of Novel Plant-Derived Antibody-Based Therapeutic Molecules against West Nile Virus. PLoS ONE, vol. 9, no. 3, pp. e93541 DOI:93510.91371/journal.pone.0093541, 2014
- H Lai, J He, J Hurtado, J Stahnke, A Fuchs, E Mehlhop, S Gorlatov, A Loos, MS Diamond and Q Chen. Structural and functional characterization of an anti-West Nile virus monoclonal antibody and its single-chain variant produced in glycoengineered plants. Plant Biotechnology Journal, 2014; vol. 12, no. 8, pp. 1098-1107, 2014
- J He, H Lai, C Brock and Q Chen. A Novel System for Rapid and Cost-Effective Production of Detection and Diagnostic Reagents of West Nile Virus in Plants. Journal of Biomedicine and Biotechnology, 2012; vol. 2012, no. 10.1155/2012/106783, pp. 1-10
- H Lai, M Engle, A Fuchs, T Keller, S Johnson, S Gorlatov, MS Diamond and Q Chen. Monoclonal antibody produced in plants efficiently treats West Nile virus infection in mice. Proceedings of the National Academy of Sciences of the United States of America, 2010; vol. 107, no. 6, pp. 2419-2424
- C Arntzen. Plant-made pharmaceuticals: from ‘Edible Vaccines’ to Ebola therapeutics. Plant Biotechnology Journal. 2015; vol. 13, no. 8, pp. 1013-1016
- H Lai, J He, M Engle, MS Diamond & Q Chen. Robust production of virus-like particles and monoclonal antibodies with geminiviral replicon vectors in lettuce. Plant Biotechnology Journal, 2012; vol. 10, no. 1, pp. 95-104
- A Fulton, H Lai, Q Chen and C Zhang. Purification of monoclonal antibody against Ebola GP1 protein expressed in Nicotiana benthamiana. Journal of Chromatography A. 2015; vol. 1389, no. 0, pp. 128-132
- L Zeitlin, J Pettitt, C Scully, N Bohorova, D Kim, M Pauly, A Hiatt, L Ngo, H Steinkellner, KJ Whaley & GG Olinger. Enhanced potency of a fucose-free monoclonal antibody being developed as an Ebola virus immunoprotectant. Proceedings of the National Academy of Sciences of the United States of America. 2011; vol. 108, no. 51, pp. 20690-20694.
- W Phoolcharoen, JM Dye, J Kilbourne, K Piensook, WD Pratt, CJ Arntzen, Q Chen, HS Mason and MM Herbst-Kralovetz. A nonreplicating subunit vaccine protects mice against lethal Ebola virus challenge. Proceedings of the National Academy of Sciences of the United States of America. 2011; vol. 108, no. 51, pp. 20695-20700
- W Phoolcharoen, SH Bhoo, H Lai, J Ma, CJ Arntzen, Q Chen and HS Mason. Expression of an immunogenic Ebola immune complex in Nicotiana benthamiana. Plant Biotechnology Journal. 2011; vol. 9, no. 7, pp. 807-816
- GG Olinger, J Pettitt, D Kim, C Working, O Bohorov, B Bratcher, E Hiatt, SD Hume, A K Johnson, J Morton, M Pauly, KJ Whaley, CM Lear, JE Biggins, C Scully, L Hensley & L Zeitlin. Delayed treatment of Ebola virus infection with plant-derived monoclonal antibodies provides protection in rhesus macaques. Proceedings of the National Academy of Sciences of the United States of America, 2012
- X Qiu, G Wong, J Audet, A Bello, L Fernando, JB Alimonti, H Fausther-Bovendo, H Wei, J Aviles, E Hiatt, A Johnson, J Morton, K Swope, O Bohorov, N Bohorova, C Goodman, D Kim, MH Pauly, J Velasco, J Pettitt, GG Olinger, K Whaley, B Xu, JE Strong, L Zeitlin and GP Kobinger. Reversion of advanced Ebola virus disease in nonhuman primates with ZMapp. Nature, 2014; vol. 514, no. 7520, pp. 47-53
- AA McCormick, MH Kumagai, K Hanley, TH Turpen, I Hakim, LK Grill, D Tuse, S Levy and R Levy. Rapid production of specific vaccines for lymphoma by expression of the tumor-derived single-chain Fv epitopes in tobacco plants. Proceedings of the National Academy of Sciences of the United States of America, 1999, vol. 96, no. 2, pp. 703-708
- AA McCormick, SJ Reinl, TI Cameron, F Vojdani, M Fronefield, R Levy and D Tuse. Individualized human scFv vaccines produced in plants: humoral anti-idiotype responses in vaccinated mice confirm relevance to the tumor Ig. Journal of Immunological Methods. 2003; vol. 278, no. 1-2, pp. 95-104
- AA McCormick, S Reddy, SJ Reinl, TI Cameron, DK Czerwinkski, F Vojdani, KM Hanley, SJ Garger, EL White, J Novak, J Barrett, RB Holtz, D Tusé & R Levy. Plant-produced idiotype vaccines for the treatment of non-Hodgkin’s lymphoma: Safety and immunogenicity in a phase I clinical study. Proceedings of the National Academy of Sciences of the United States of America. 2008; vol. 105, no. 29, pp. 10131-10136
- DM Morens. Antibody-dependent of enhancement of infection and the pathogenesis of viral disease. Clin Inf Dis. 1994; vol. 19, pp. 500-512
- Q Chen & H Lai. Plant-derived virus-like particles as vaccines,” Human Vaccines & Immunotherapeutics, 2013; vol. 9, no. 1, pp. 26-49
- K Traynor. Taliglucerase alfa approved for Gaucher disease. Am J Health Syst Pharm. 2012; vol. 69, no. 12, pp. 1009
- BR Holtz, BR Berquist, LD Bennett, VJM Kommineni, RK Munigunti, EL White, DC Wilkerson, K-YI Wong, LH Ly & S. Marcel. Commercial-scale biotherapeutics manufacturing facility for plant-made pharmaceuticals. Plant Biotechnology Journal. 2015; vol. 13, no. 8, pp. 1180-1190
- JKC Ma, J Drossard, D Lewis, F Altmann, J Boyle, P Christou, T Cole, P Dale, CJ van Dolleweerd, V Isitt, D Katinger, M Lobedan, H Mertens, MJ Paul, T Rademacher, M Sack, PAC Hundleby, G Stiegler, E Stoger, RM Twyman, B Vcelar & R Fischer. Regulatory approval and a first-in-human phase I clinical trial of a monoclonal antibody produced in transgenic tobacco plants. Plant Biotechnology Journal. 2015; vol. 13, no. 8, pp. 1106-1120
- H Lai & Q Chen. Bioprocessing of plant-derived virus-like particles of Norwalk virus capsid protein under current Good Manufacture Practice regulations. Plant Cell Reports. 2012; vol. 31, no. 3, pp. 573-584
Biographies
Dr. Qiang “Shawn” Chen, PhD, is a Professor in the Center for Infectious Diseases at Arizona State University. Dr. Chen spent more than ten years in the biotechnology and pharmaceutical industry directing research in therapeutic protein development in both plant and mammalian cell culture systems. Prior to joining ASU, Dr. Chen was the Associate Director of the Division of Protein Chemistry at Cardinal Health and a Sr. Scientist at Monsanto. Email Dr. Chen at: [email protected];
Huafang Lai is a research scientist in Dr. Chen’s laboratory. Their lab focuses on optimising the expression and assembly of monoclonal antibodies and designing novel mAb fusion proteins to enhance their targeting and efficacy. Email Huafang at: huafang.lai@ asu.edu
Related topics
Antibody Discovery, Drug Delivery, Protein Expression
Related conditions
Ebola
Related organisations
University of Arizona
Related people
Huafang Lai, Qiang “Shawn” Chen