New system for safer CRISPR-based gene drive testing and development
Posted: 20 January 2023 | Izzy Wood (Drug Target Review) | No comments yet
US researchers outline the development of a new system for testing and developing CRISPR-based gene drives in the laboratory, and safely converting them into tools for potential real-world applications.
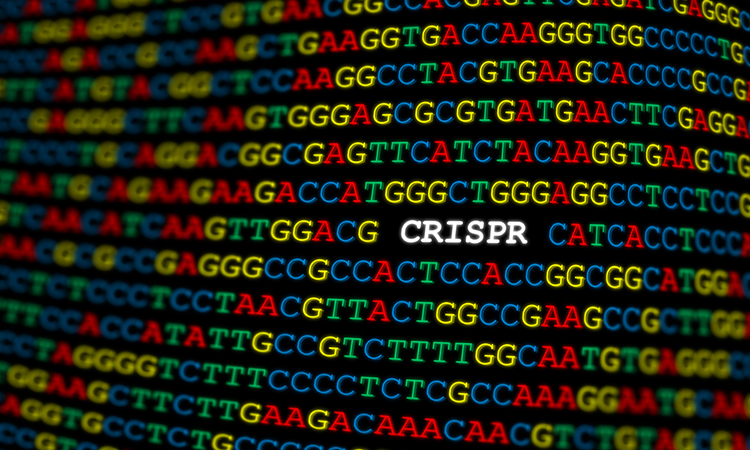
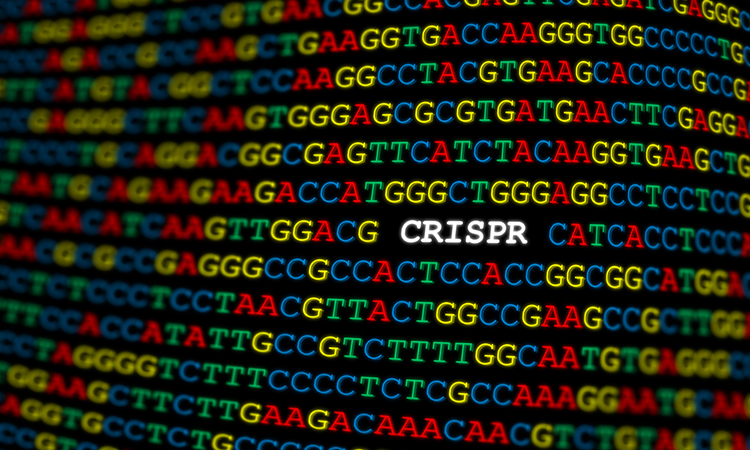
University of California (UC), US, scientists continue to expand the technological frontiers of CRISPR, along with CRISPR-based gene drives, a genetic editing tool designed to influence how genetic elements are passed from one generation to the next.
The research, published in Nature Communications, outlines the development of a new system for testing and developing gene drives in the laboratory and safely converting them into tools for potential real-world applications.
Gene drives designed for mosquitoes have the potential to curb the spread of malarial infections, yet safety issues have been raised since such drives can spread quickly and dominate entire populations. Scientists have explored the principles governing the spread of gene-drive elements in targeted populations such as mosquitoes by testing many different combinations of components that constitute the drive apparatus.
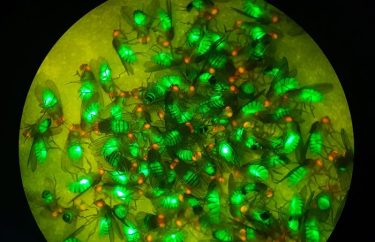
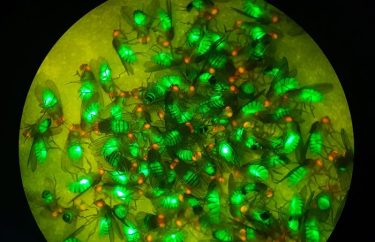
Fluorescent Flies: Fruit flies expressing red eye and green body fluorescent markers demonstrated the viability of a new system for safely converting split gene drives into full gene drives(Credit: Bier Lab, UC San Diego)
“These studies both empower new engineering of gene-drive systems while providing important information regarding how to assess and analyse key interactions between their most important moving parts,” said Professor Ethan Bier, a faculty member in the School of Biological Sciences, Department of Cell and Developmental Biology.
CRISPR-based gene drives feature a protein called a Cas9 endonuclease and a guide RNA molecule that join forces to direct DNA cuts to specific sites in the genome where new genetic elements can be inserted. As the DNA repairs these cuts, the new genetic elements are copied from one chromosome to another, resulting in offspring that exceed the standard 50-50 percent inheritance, instead favouring the newly inserted genetic elements.
There are two types of gene drives: full gene drives (fGDs), that carry both the Cas9 and guide RNA components in a linked unitary package. In contrast, split drives (sGDs) consist of two genetic elements, that separately carry the Cas9 and guide RNA components and are inserted at different sites in the genome.
sGDs are considered to be safer than fGDs since it is possible to control and test the components carried by each of the elements separately or under conditions where they gradually amplify the frequency of the gRNA component. Researchers design the two elements to eventually reconnect in order to deliver the effects of a full gene drive.
In the case of eradicating malaria, full gene drives have created considerable enthusiasm due to their potential as vehicles to transfer elements that halt the transmission of malarial parasites that cause infection.
However, fGDs have also raised concerns due to their potential to rapidly spread and potentially alter the genetic makeup of entire mosquito populations. Experimenting with fGDs requires high-security barriers and restrictions to prevent unintended escape of insects carrying such drives into the open environment.
This is not the case with split gene drives because the key elements are separate. sGDs carry far less risk of unintentional spread, and experiments with sGDs can be conducted in traditional lab facilities, thus allowing much more flexibility for testing their potential.
Now, UC scientists who have recently pioneered gene drive development and related technologies have created a flexible genetic “hacking” system for converting sGDs into fGDs.
Working in fruit flies, the researchers developed a novel genetic strategy that employs a specially designed guide RNA carried by the Cas9 part of the sGD. This hacking tool cuts the copying component of the sGD and triggers a genetic exchange that inserts the Cas9 into the element carrying the guide RNA, resulting in the creation of a fully functioning fGD.
The newly hacked fGD spread through populations of flies in cage experiments, as expected. However, the rate at which it spreads was unexpectedly slower than models had predicted for a traditional fGD.
Thus, the team developed a mathematical model that provided an explanation. Their model revealed that during the hacking conversion, fGDs impose a greater fitness cost on individual flies than sGDs alone. This fitness cost, which unfolds when the drive element copies itself, vanished after acting on all potential target chromosomes in the population.
“The study revealed unanticipated complexities in how gene-drive components work together, showing that one cannot simply assume how separate components may interact when brought together,” concluded UC Berkeley graduate student Jared Bennett.
Related topics
CRISPR, DNA, Drug Development, Genetic Analysis, RNAs, Targets, Therapeutics
Related conditions
Malaria
Related organisations
University of California (UC)
Related people
Jared Bennett, Professor Ethan Bier