Genomics in drug development and safety testing; from target identification to genomic toxicology
Posted: 3 July 2018 | Adam David Thomas | No comments yet
In the annual report of the Chief Medical Officer, 1 Dame Sally Davies has declared that we are part of ‘generation genome’ – the era in which we reap the rewards of our advances in genomic technologies and improvements in our understanding of the whole genome in human health.
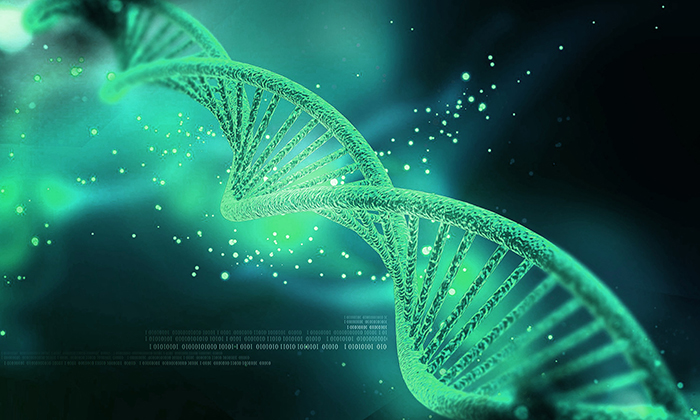
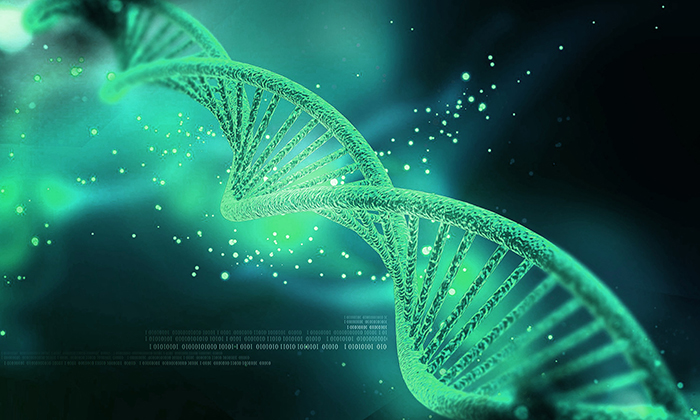
This article provides a review of the positive impact genomics can have on drug discovery, development and deployment in medicine.
We are only just beginning to realise the beneficial impact of genomics on human health, which was perhaps prematurely promised at the advent of the human genome project. Aided by the advancement in high-throughput (next generation) whole genome sequencing technologies and a simultaneous reduction in cost, the application of genomic technologies is becoming widespread. For example, genomics is becoming integrated into routine clinical practice, so-called genomic medicine. In the UK, the 100,000 genome project, led by Genome England, is a flagship project that is driving the ‘mainstreaming’ of genomics in the NHS, the benefits of which are already apparent in the diagnosis and clinical management of individuals.2 The big data that will be generated by these, and other, projects that employ genome-wide association studies (GWAS), microarray, whole genome (WGS) and whole exome sequencing (WES) approaches on vast numbers of patients will have far wider implications. For example, in target identification and drug development, as we further our understanding of genetic profiles underlying disease; while the high-throughput and cheap sequencing technologies could play a role in drug safety testing.
Genomics in target identification
Genomics offers a new resource for therapeutic opportunity by identifying disease associations and targets for intervention. Our focus has been concentrated on the druggable genome; that is, the genes that encode proteins able to bind small molecules.3 This prediction is based on sequence and structural homology to known drug targets. There are many databases, such as ‘The Drug Gene Interaction Database’, that offer a wealth of publicly-available genome information to mine and identify the genes of potential therapeutic targets.4 What is defined as the druggable genome is ever increasing. It is currently estimated that 4,479 genes fall into this category and offer therapeutic opportunities for new or repurposed drugs.5 Does this mean that the remaining 88% of protein-coding genes in the human genome is undruggable, or yet to be defined as amenable to intervention? Of the 4,479 druggable genes, our knowledge of one third of these genes is lacking. This knowledge gap was identified by a three-year pilot study by the National Institutes of Health (NIH) and its Common fund ‘Illuminating the Druggable Genome’ (IDG) project.6 Furthermore, the vast majority of the human genome (98%) has unknown function. Mislabelled as ‘junk’ DNA, it is becoming evident that this cannot be ignored. Homage needs to be paid to the National Human Genome Research Institute’s (NHGRI’s) funded encyclopaedia of DNA Elements (ENCODE) project7 that aims to assign functionality to the unknown genome.
With the benefit of whole genome sequencing and fundamental research into functional genomics (linking DNA to protein function), we are beginning to understand the relevance of unexplored regions of the druggable genome but also the entirety of genomic DNA for the benefit of basic human biology, drug development and even drug approval. It is calculated that targets supported by genetic data (where genes have a causal link to disease) have double the success rate in clinical development.8 This has been attributed to selecting the best ‘gene-drug pair’ based on genetic information.9 The potential benefit of genomic information in drug approval is evident.
Genomic approaches to safety testing
The safety assessment of novel drugs involves a standardised battery of in vitro and in vivo assays to characterise mutagenic and carcinogenic potential.10 The current battery of tests have been in use for many years but there is a real opportunity to extend the scope of these assays to include analysis of the whole genome. The Genetic Toxicology Technical Committee (GTTC) of the International Life Sciences Institute (ILSI) Health and Environmental Sciences Institute (HESI) held workshops at recent meetings to consider a ‘next generation’ testing strategy to address, amongst other matters, “the need to expand from genetic to genomic alterations, and to include other endpoints of genomic damage associated with human diseases besides cancer”.11 Of course, this is applicable to whole genome sequencing approaches but also the analysis of other ‘omics’. Incorporating follow-up genomic approaches to existing in vitro and in vivo mutation assays will draw further information from existing tests, allow assessment of the mutational load across the genome following exposure, and, importantly, provide a mutational signature to help inform a mechanism of action and cell specificities. This will aid mechanistic / investigative or predictive genomic toxicology. Genomics in drug approval The primary aim of sequencing the whole patient genome is to personalise future treatment decisions and such an approach is being used in clinical trial design and deployment. The most widely seen are genotyping arrays 12 in oncology where drug selection is based upon the mutation profile (biomarker) of the cancer.13 An example is seen with AstraZeneca’s recent phase III FLAURA trial of TAGRISSO (osimertinib), specifically deployed against epidermal growth factor receptor (EGFR)‑mutated lung cancer. Thus improving those prospects being treated, but also aiding regulatory approval. Furthermore, genomic screening could help stratify patients into subgroups by their pharmacogenomics, ie, identify those who are more likely to benefit from treatment and exclude those likely to suffer adverse drug reactions.14 Of course, this is based on fundamental genomic research at prior in vitro, pre-clinical stages.15 To this end, follow-up genomic profiling of patients enrolled in clinical trials could yield mechanistic data, pharmacodynamics, pharmacokinetics and the genotypes of super-responders for future precision medicine decisions. Targeting the undruggable with genome editing technologies Small, polar molecules or therapeutic proteins (eg, antibodies) are effective therapeutics that can target disease-specific mutations in the genome that manifest as altered proteins. However, whole genome sequencing may reveal disease-causing variants in non-coding regions of the DNA that may not manifest themselves as alterations to proteins but may instead play important regulatory functions.
Such alterations may not be amenable to direct intervention. However, genome editing may prove an effective strategy in reversing those mutations.16 As these therapies become further developed and enter safety assessment, genomic approaches in safety assessment may prove to be invaluable in assessing the off-target effects of such technologies.
Biography
ADAM DAVID THOMAS is a Senior Lecturer in human genetics and genomics at the University of the West of England in Bristol, UK. Previously, Adam held positions at Covance laboratories, Harrogate, UK and, following his PhD award from Swansea University, Wales, UK, carried out postdoctoral work at the Institut für Toxikologie in Mainz, Germany. His research focuses on DNA damage and 3D image reconstruction of new generation genotoxicology models.
References
- Davies SC. Annual Report of the Chief Medical Officer 2016. London Department Heal. 2017.
https://www.gov.uk/government/uploads/system/uploads/attachment_data/file/631043/
CMO_annual_report_generation_genome.pdf. - Brittain HK, Scott R, Thomas E. The rise of the genome and personalised medicine. Clin Med J
R Coll Physicians London. 2017;17(6):545-551. doi:10.7861/clinmedicine.17-6-545. - Hopkins AL, Groom CR. The druggable genome. Nat Rev Drug Discov. 2002;1(9):727-730.
doi:10.1038/nrd892. - Griffith M, Griffith O, Coffman A, et al. DGIdb – Mining the druggable genome. Nat Methods.
2013;10(12):1209-1210. doi:10.1038/nmeth.2689.DGIdb. - Finan C, Gaulton A, Kruger FA, et al. The druggable genome and support for target
identification and validation in drug development. Sci Transl Med. 2017;9(383):1-40.
doi:10.1126/scitranslmed.aag1166. - Oprea TI, Bologa CG, Brunak S, et al. Unexplored therapeutic opportunities in the human
genome. Nat Rev Drug Discov. 2018;17(5):317-332. doi:10.1038/nrd.2018.14. - Encode Consortium, Carolina N, Hill C. An Integrated Encyclopedia of DNA Elements in the
Human Genome. Nature. 2013;489(7414):57-74. doi:10.1038/nature11247.An. - Nelson MR, Tipney H, Painter JL, et al. The support of human genetic evidence for approved
drug indications. Nat Genet. 2015;47(8):856-860. doi:10.1038/ng.3314. - Plenge RM, Scolnick EM, Altshuler D. Validating therapeutic targets through human genetics.
Nat Rev Drug Discov. 2013;12(8):581-594. doi:10.1038/nrd4051. - Eastmond DA, Hartwig A, Anderson D, et al. Mutagenicity testing for chemical risk assessment:
Update of the WHO/IPCS Harmonized Scheme. Mutagenesis. 2009;24(4):341-349. doi:10.1093/
mutage/gep014. - Dearfield K, Gallopudi B, Bemis J, et al. In-silico design, synthesis and anti-proliferative
evaluation of acetidino-quinazoline derivatives. Environ Mol Mutagen. 2017;58:264-283.
doi:10.1002/em. - Monte A, Vasiliou V, Heard K. Omics Screening for Pharmaceutical Efficacy and Safety in
Clinical Practice. J Pharmacogenomics Pharmacoproteomics. 2012;16(s5). doi:10.1109/
TMI.2012.2196707.Separate. - Simon R, Roychowdhury S. Implementing personalized cancer genomics in clinical trials. Nat
Rev Drug Discov. 2013;12(5):358-369. doi:10.1038/nrd3979. - Raja R, Lee YS, Streicher K, et al. Integrating Genomics into Drug Discovery and Development:
Challenges and Aspirations. Pharmaceut Med. 2017;31(4):217-233. doi:10.1007/s40290-017-
0192-8. - Perez-Gracia JL, Sanmamed MF, Bosch A, et al. Strategies to design clinical studies to identify
predictive biomarkers in cancer research. Cancer Treat Rev. 2017;53:79-97. doi:10.1016/j.
ctrv.2016.12.005. - Gaudelli NM, Komor AC, Rees HA, et al. Programmable base editing of A • T to G • C in
genomic DNA without DNA cleavage. Nat Publ Gr. 2017;551(7681):464-471. doi:10.1038/
nature24644.
The rest of this content is restricted - login or subscribe free to access
Why subscribe? Join our growing community of thousands of industry professionals and gain access to:
- quarterly issues in print and/or digital format
- case studies, whitepapers, webinars and industry-leading content
- breaking news and features
- our extensive online archive of thousands of articles and years of past issues
- ...And it's all free!
Click here to Subscribe today Login here
Related topics
DNA, Drug Development, Drug Discovery, Drug Discovery Processes, Genomics, In Vitro, In Vivo, Sequencing, Small molecule, Toxicology
Related organisations
BD, NHS, University of the West of England (UWE)
Related people
Adam David Thomas