Application of organoid technology for retinal disease modelling and drug discovery
Posted: 4 June 2020 | Valeria Chichagova (Newcastle University and Newcells Biotech) | No comments yet
The loss of retinal light-sensing photoreceptor cells is a leading cause of blindness and the number of individuals affected by retinal degenerative diseases is increasing with an ageing population. Currently, there are no treatments for these diseases and progress in finding new treatments is slow. This article explores the potential of human pluripotent stem cell-derived retinal organoids as a platform for disease modelling and to enable faster and more efficient drug discovery processes.
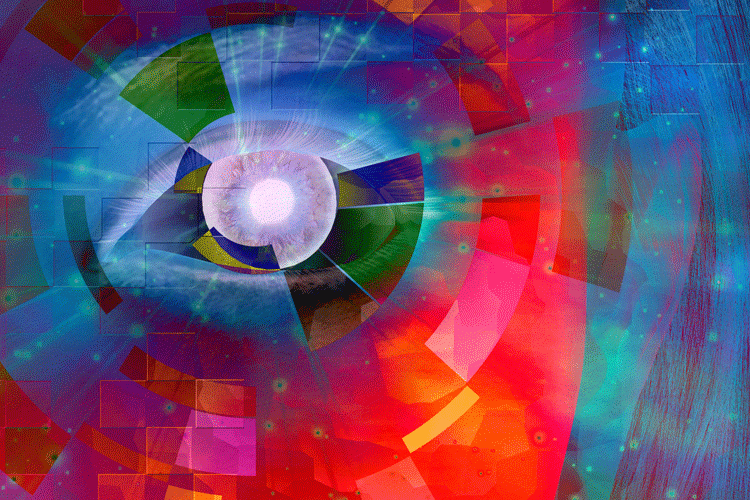
Globally, the number of people with mild-to-moderate visual impairment is estimated to be 217 million, with an additional 36 million registered as blind. While some cases stem from a lack of access to treatment, many result from a range of untreatable conditions.1 In the UK alone, total annual healthcare costs associated with visual problems amount to £2 billion and in the US this number is a staggering $51.4 billion.2,3 The impact of vision loss is expected to increase with an ageing population; putting additional social and economic burdens on societies, making the prevention and treatment of vision loss a public health priority.
The most common ocular diseases affecting individuals in the developed world are those that result in the degeneration of the retina. The human retina is a complex multicellular tissue located at the back of the eye, consisting of stratified neurosensory retina and an underlying layer of supporting retinal pigment epithelium (RPE) cells. The neural retina is made up of five major cell types, including photoreceptors, bipolar, horizontal, amacrine and retinal ganglion cells (RGCs), of which most contain multiple subtypes. Photoreceptors are key for converting light energy into an electrical signal via a cascade of biochemical reactions, resulting in an action potential that is transmitted to the brain for image interpretation. RPE cells are critical for maintaining homeostasis of photoreceptors and form a blood-retinal barrier. Other retinal cell types, such as glial cells, provide structural and metabolic support or are involved in the signalling cascade between the photoreceptors and brain.4 When a component of this intricate network is compromised, it has a downstream effect on the rest of the retina and as a result leads to retinal degeneration and a compromised functional output.
Retinal degeneration may be a result of various environmental and genetic factors. Age-related macular degeneration (AMD) is the leading cause of blindness in elderly patients in developed countries. RPE is a primary site of pathology in AMD, which causes the degeneration of the macula, a part of the retina that is responsible for central vision. The main risk factors in developing this disease are age, environmental factors, lifestyle choices (such as diet and smoking) and hereditary predisposition.5,6 Another group of conditions have a genetic component and are referred to as inherited retinal diseases (IRDs); the main contributor to visual impairment amongst working-age individuals. Retinitis pigmentosa (RP) is the most common IRD with an approximate prevalence of 1:3,500-5,000.7 It embraces a group of retinal disorders characterised by progressive retinal degeneration, resulting in night blindness, loss of peripheral vision and eventual blindness. There are currently no effective treatments for inherited retinal degeneration so there is a requirement for model systems that would enable the study of this disease’s mechanisms in the context of human physiology. Most of our knowledge on the retina in health and disease has been based on studies performed using various cellular and animal models. Ocular research has benefited significantly from the use of rodent models which, despite numerous advantages, have several limitations driven largely by the differences in lifespan and physiology compared to humans, including a lack of the macula – a region responsible for fine vision – and reliance on night-time vision.8 For some pathological processes, no animal models currently exist. In human studies, access to post-mortem tissues is limited with the available tissue often representing the end stage of the disease, whereas cell models of individual retinal cell types have insufficient complexity of cell-cell interaction. Lack of adequate models has therefore been the driving force for the development of in vitro retinal models based on human induced pluripotent stem cells (hiPSCs).
Several studies have used retinal organoid technology to elucidate disease mechanisms and offer potential future therapeutic approaches”
Human iPSCs were discovered in 2007 and have had a profound effect on various areas of science.9,10 They are derived by reprogramming terminally differentiated cells into an embryonic‑like pluripotent state, lending them their defining features. They are characterised by indefinite proliferation and multilineage differentiation, properties found in human embryonic stem cells (hESCs), though without the ethical implications of using cells from pre-implantation embryos. Generating hiPSCs from patient cells provides an unlimited source for creating clinically relevant platforms that can display pathogenesis of retinal disease.
Several studies have used retinal organoid technology to elucidate disease mechanisms and offer potential future therapeutic approaches. Leber congenital amaurosis (LCA) is an inherited retinal dystrophy with a prevalence of approximately 1:50,000 and accounts for five percent of all IRDs.23 It is characterised by severe visual impairment from birth with photoreceptor degeneration attributed as the main cause of blindness. Mutations in a cilia-related gene, CEP290, account for between six and 22 percent of cases, with the most common variant causing mis-splicing and premature termination.24 A recent study used LCA-patient derived hiPSCs to generate RPE and retinal organoids for the study of disease mechanisms and a potential therapeutic approach,25 allowing the authors to gain a mechanistic insight into the disease processes causing the retina-specific phenotype. They also demonstrated the potential of antisense oligonucleotides as a possible therapeutic strategy by showing the rescue of disease phenotype. Another study used cells from RP patients with mutations in one of the most prevalent causative genes, RPGR, to generate retinal organoids and RPE cells.26 They were able to demonstrate the pathological phenotype displayed by photoreceptors and RPE cells. As a proof-of-concept, they used CRISPR-Cas9-mediated gene correction and showed rescued photoreceptor structure and function as well as restoration of gene expression levels. A different study investigated the mechanisms of autosomal dominant RP caused by mutations in pre-mRNA processing factor 31 (PRPF31) by also harnessing the power of organoid technology.27 Mis-splicing of genes implicated in ciliogenesis and adhesion resulted in patient-derived retinal organoids and RPE displaying disrupted cilia morphology. In addition, RPE cells had disrupted apical-basal polarity and reduced functional output. In situ gene editing using a CRISPR-Cas9 approach rescued key phenotypes and provided proof-of-concept for future therapeutic applications. Retinal organoids have also been shown as a valuable platform for studying gene delivery systems such as vectors based on adeno‑associated virus (AAV), which would enable the prediction of outcomes in human gene therapy.28Early protocols for generating retinal cells from human pluripotent stem cells (hPSCs) relied on a two-dimensional (2D) approach that lacked the complexity of retinal tissue.11,12 These were followed by reports that described the generation of more complex three-dimensional (3D) structures.13,14 The pioneering work by Yoshiki Sasai’s group was the first to describe the generation of laminated 3D retinal organoids that closely mimicked the developmental timeline of human retinogenesis and contained stratified retina.15 Other approaches and further modifications resulted in the generation of improved retinal organoids that displayed more mature phenotypes, including the formation of outer segments, synapses and the ability for phototransduction.16-22 Due to their increased complexity, retinal organoids are becoming an emerging platform for disease modelling in the drug discovery process.
The development of retinal organoid technology has greatly improved access to human retinal tissue”
Retinal organoids have been used in other studies aiming to phenotypically characterise the effect of disease-causing mutations. Guo et al. showed that retinal organoids derived from RP patients with the mutation in the USH2A gene presented early developmental abnormalities.29 A model of late-onset RP has been established, harbouring a mutation in PDE6B.30 Retinal organoids were shown to have normal development, however at later stages of maturation they remarkably recapitulated the disease phenotype associated with impaired photoreceptor maturation.
In addition to their applicability in the study of the disease mechanisms and investigating novel therapeutic approaches, retinal organoids have also been utilised for drug screening purposes. Hallam et al. generated functional light-responsive retinal organoids and treated them with a known retinotoxin moxifloxacin.31 The authors showed a similar response to the drug when compared to published in vivo data for mouse adult retina indicating that the photoreceptors were the primary-affected phenotype. Therefore, this study demonstrated the potential applicability of the model for drug screening purposes.
The development of retinal organoid technology has greatly improved access to human retinal tissue. We are now able to generate an unlimited number of retinal organoids to study human development, disease progression and potential new therapies. Retinal organoid technology is a physiologically relevant model of the human retina which has the potential to revolutionise the approach to developing new treatments for retinal diseases.
About the author
After graduating from the University of Edinburgh, Valeria Chichagova moved to Newcastle University to pursue a PhD investigating the effect of a common mitochondrial DNA mutation on the development of pigmentary retinopathy using patient-derived induced pluripotent stem cells (hiPSCs) as a model. She then moved on to do a postdoc at Newcastle University looking at disease mechanisms of retinitis pigmentosa using retinal organoids derived from patient hiPSCs. Valeria is an Innovation Manager at Newcells Biotech, delivering assays for toxicology and drug discovery using hiPSC technology and an Honorary Associate Fellow at Newcastle University.
References
1. Bourne RRA, Flaxman SR, Braithwaite T, Cicinelli MV, Das A, Jonas JB, et al. Magnitude, temporal trends, and projections of the global prevalence of blindness and distance and near vision impairment: a systematic review and meta-analysis. Lancet Glob Health. 2017;5(9):e888-e97.
2. RNIB. Key information and statistics on sight loss in the UK 2019 [Available from: https://www.rnib.org.uk/professionals/…
3. CDC. Vision Health Initiative – Economic Stidues 2017 [Available from: https://www.cdc.gov/visionhealth/projects/economic_studies.htm.
4. Grossniklaus HE, Geisert EE, Nickerson JM. Introduction to the Retina. Progress in molecular biology and translational science. 2015;134:383-96.
5. Mitchell P, Liew G, Gopinath B, Wong TY. Age-related macular degeneration. Lancet (London, England). 2018;392(10153):1147-59.
6. Chichagova V, Hallam D, Collin J, Zerti D, Dorgau B, Felemban M, et al. Cellular regeneration strategies for macular degeneration: past, present and future. Eye (Lond). 2018;32(5):946-71.
7. Sorrentino FS, Gallenga CE, Bonifazzi C, Perri P. A challenge to the striking genotypic heterogeneity of retinitis pigmentosa: a better understanding of the pathophysiology using the newest genetic strategies. Eye (Lond). 2016;30(12):1542-8.
8. Mustafi D, Kevany BM, Bai X, Golczak M, Adams MD, Wynshaw-Boris A, et al. Transcriptome analysis reveals rod/cone photoreceptor specific signatures across mammalian retinas. Hum Mol Genet. 2016;25(20):4376-88.
9. Yu J, Vodyanik MA, Smuga-Otto K, Antosiewicz-Bourget J, Frane JL, Tian S, et al. Induced pluripotent stem cell lines derived from human somatic cells. Science (New York, NY). 2007;318(5858):1917-20.
10. Takahashi K, Tanabe K, Ohnuki M, Narita M, Ichisaka T, Tomoda K, et al. Induction of pluripotent stem cells from adult human fibroblasts by defined factors. Cell. 2007;131(5):861-72.
11. Jin ZB, Okamoto S, Xiang P, Takahashi M. Integration-free induced pluripotent stem cells derived from retinitis pigmentosa patient for disease modeling. Stem cells translational medicine. 2012;1(6):503-9.
12. Jin ZB, Okamoto S, Osakada F, Homma K, Assawachananont J, Hirami Y, et al. Modeling retinal degeneration using patient-specific induced pluripotent stem cells. PloS one. 2011;6(2):e17084.
13. Meyer JS, Howden SE, Wallace KA, Verhoeven AD, Wright LS, Capowski EE, et al. Optic vesicle-like structures derived from human pluripotent stem cells facilitate a customized approach to retinal disease treatment. Stem Cells. 2011;29(8):1206-18.
14. Phillips MJ, Wallace KA, Dickerson SJ, Miller MJ, Verhoeven AD, Martin JM, et al. Blood-derived human iPS cells generate optic vesicle-like structures with the capacity to form retinal laminae and develop synapses. Investigative ophthalmology & visual science. 2012;53(4):2007-19.
15. Nakano T, Ando S, Takata N, Kawada M, Muguruma K, Sekiguchi K, et al. Self-formation of optic cups and storable stratified neural retina from human ESCs. Cell stem cell. 2012;10(6):771-85.
16. Reichman S, Terray A, Slembrouck A, Nanteau C, Orieux G, Habeler W, et al. From confluent human iPS cells to self-forming neural retina and retinal pigmented epithelium. Proceedings of the National Academy of Sciences of the United States of America. 2014;111(23):8518-23.
17. Zhong X, Gutierrez C, Xue T, Hampton C, Vergara MN, Cao LH, et al. Generation of three-dimensional retinal tissue with functional photoreceptors from human iPSCs. Nat Commun. 2014;5:4047.
18. Wahlin KJ, Maruotti JA, Sripathi SR, Ball J, Angueyra JM, Kim C, et al. Photoreceptor Outer Segment-like Structures in Long-Term 3D Retinas from Human Pluripotent Stem Cells. Sci Rep. 2017;7(1):766.
19. Kuwahara A, Ozone C, Nakano T, Saito K, Eiraku M, Sasai Y. Generation of a ciliary margin-like stem cell niche from self-organizing human retinal tissue. Nat Commun. 2015;6:6286.
20. Dorgau B, Felemban M, Hilgen G, Kiening M, Zerti D, Hunt NC, et al. Decellularised extracellular matrix-derived peptides from neural retina and retinal pigment epithelium enhance the expression of synaptic markers and light responsiveness of human pluripotent stem cell derived retinal organoids. Biomaterials. 2019;199:63-75.
21. Zerti D, Dorgau B, Felemban M, Ghareeb AE, Yu M, Ding Y, et al. Developing a simple method to enhance the generation of cone and rod photoreceptors in pluripotent stem cell-derived retinal organoids. Stem Cells. 2020;38(1):45-51.
22. Ovando-Roche P, West EL, Branch MJ, Sampson RD, Fernando M, Munro P, et al. Use of bioreactors for culturing human retinal organoids improves photoreceptor yields. Stem cell research & therapy. 2018;9(1):156.
23. Koenekoop RK. An overview of Leber congenital amaurosis: a model to understand human retinal development. Survey of ophthalmology. 2004;49(4):379-98.
24. Chacon-Camacho OF, Zenteno JC. Review and update on the molecular basis of Leber congenital amaurosis. World J Clin Cases. 2015;3(2):112-24.
25. Parfitt DA, Lane A, Ramsden CM, Carr AJ, Munro PM, Jovanovic K, et al. Identification and Correction of Mechanisms Underlying Inherited Blindness in Human iPSC-Derived Optic Cups. Cell stem cell. 2016;18(6):769-81.
26. Deng WL, Gao ML, Lei XL, Lv JN, Zhao H, He KW, et al. Gene Correction Reverses Ciliopathy and Photoreceptor Loss in iPSC-Derived Retinal Organoids from Retinitis Pigmentosa Patients. Stem cell reports. 2018;10(4):1267-81.
27. Buskin A, Zhu L, Chichagova V, Basu B, Mozaffari-Jovin S, Dolan D, et al. Disrupted alternative splicing for genes implicated in splicing and ciliogenesis causes PRPF31 retinitis pigmentosa. Nat Commun. 2018;9(1):4234.
28. Garita-Hernandez M, Routet F, Guibbal L, Khabou H, Toualbi L, Riancho L, et al. AAV-Mediated Gene Delivery to 3D Retinal Organoids Derived from Human Induced Pluripotent Stem Cells. International journal of molecular sciences. 2020;21(3).
29. Guo Y, Wang P, Ma JH, Cui Z, Yu Q, Liu S, et al. Modeling Retinitis Pigmentosa: Retinal Organoids Generated From the iPSCs of a Patient With the USH2A Mutation Show Early Developmental Abnormalities. Frontiers in cellular neuroscience. 2019;13:361.
30. Gao ML, Lei XL, Han F, He KW, Jin SQ, Zhang YY, et al. Patient-Specific Retinal Organoids Recapitulate Disease Features of Late-Onset Retinitis Pigmentosa. Frontiers in cell and developmental biology. 2020;8:128.
31. Hallam D, Hilgen G, Dorgau B, Zhu L, Yu M, Bojic S, et al. Human-Induced Pluripotent Stem Cells Generate Light Responsive Retinal Organoids with Variable and Nutrient-Dependent.