The growing possibilities for stem cells in pharma
Posted: 28 January 2020 | Hannah Balfour (Drug Target Review) | No comments yet
Drug Target Review explores the latest applications of stem cells in modelling disease, drug production and the most recent steps in regenerative medicine provided by research.
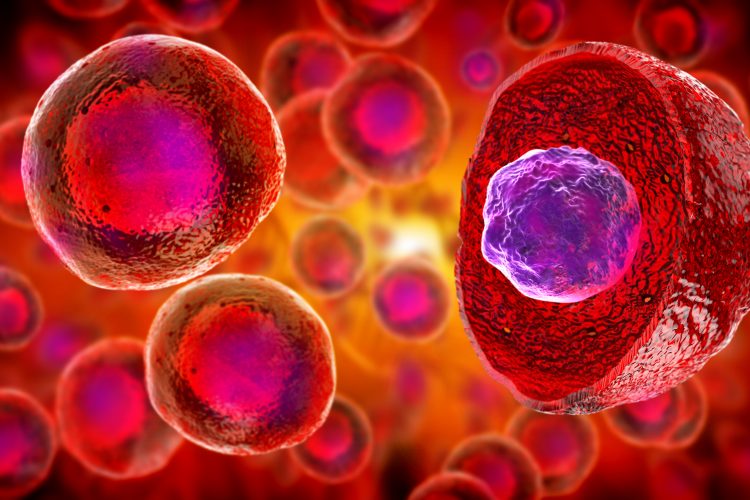
For decades, researchers have been investigating how to steer stem cell differentiation to create models and engineer tissues for various applications. The capabilities of stem cells appear endless; disease modelling, drug production and therapeutic advancements are all potential applications.
Drug Target Review’s Hannah Balfour explored the latest uses for stem cells in therapeutic R&D, to discover what these developments could provide.
Tissue models
In order to be used as an effective tissue or disease model, stem cells must be encouraged to differentiate into certain cell types. Stem cells are naturally reactive to their environment, which can be manipulated by researchers to drive differentiation in vitro.
Picking bones
One of the latest developments, published in PNAS, details a simplified process of transforming stem cells into bone.1 The researchers created a dynamic scaffold for bone production, using a polymer sheet that expands and contracts in response to temperature changes to act as an artificial muscle. A grid on the underside of the sheet was seeded with stem cells before the researchers repeatedly raised and lowered the temperature between 10°C and 37°C.
Bone models could also be used to ascertain different genes’ roles in various diseases and observe the effects of treatments”
“Our polymer actuator sheet has a so-called shape-memory function… this allows it to act like a transducer, with which we can effectively instruct the cells to do as we wish. We found that the changes in temperature, combined with the repeated stretching motion of the film was enough to encourage the stem cells to differentiate into bone cells,” explained Professor Andreas Lendlein, an author of the paper and head of the Helmholtz-Zentrum Geesthacht’s Institute of Biomaterial Science, Germany.
This development should allow researchers to more simply develop bone tissue, compared to previous protocols that required the design and use of static scaffolds to support the growing bone as the extracellular matrix would in vivo.
The team suggest this technique could be used in the treatment of extreme bone fractures. Bone models could also be used to ascertain different genes’ roles in various diseases and observe the effects of treatments targeting conditions such as osteoporosis and bone cancers.
Accidentally drawing blood
A further model came as a byproduct of leukaemia research by the University of Colorado (CU) Cancer Center. The researchers were working with haematopoietic stem cells (HSCs) in the hope of developing personalised leukaemia stem cell therapies, when they identified that deleting the MLL gene from HSCs caused them to differentiate into blood cells.2
The lab was researching MLL as it has been implicated in approximately five percent of all childhood leukaemias due to a rearrangement where it fuses with a series of other genes.
Dr Patricia Ernst, CU Cancer Center investigator and Professor in the CU School of Medicine Departments of Pediatrics, said: “When we knocked out this gene [MLL], we saw that haematopoietic stem cells couldn’t retain their ‘stemness’ – instead of being HSCs, they would differentiate to become like normal cells of the blood system. So, we wondered what would happen if we increased it.”
The findings of the study, published in Stem Cell Reports, show that doubling the amount of the regular MLL protein in HSCs drives them to differentiate into blood cells. Ernst highlighted that these cells are the first HSCs that show the potential to repopulate a patient’s immune system in vivo, following chemotherapy or radiation. This method of blood cell production could also be used in isolation to reveal how drugs and diseases target the blood function, as well as in conjunction with other models to study circulation.
Drug producers
Another possible application of stem cells is to secrete fully humanised factors. The myriad uses for these could include insulin for diabetic patients and growth hormones for growth disorders.
The healing properties of fat
One of the latest developments using stem cells as a drug producer comes from a research team at Nanyang Technological University, Singapore (NTU Singapore), which found a method to enhance adult mesenchymal stem cells (MSCs) production of healing factors.3 Using a hydrogel, the researchers grew MSCs on a surface mimicking the density of body fat and found this softer surface increased the secretion of healing factors in the MSC secretome, compared to normal growing surfaces.
MSCs are known to produce healing factors in response to oxygen starvation through the hypoxia-inducible factor 1-alpha (HIF 1-α), a major switch which, when activated, promotes tissue blood vessel growth and repair.
This study showed that tissue softness can activate HIF 1-α signalling under normal oxygen conditions, without any additional biological or pharmacological agents. The researchers hope this knowledge will result in advanced cell culture materials that are able to improve the production and therapeutic potential of MSC secretomes in future.
“Our goal is to make MSCs produce the same healing factors in the lab as they do in the body during tissue repair and that these might then be made into serums or incorporated into tissue patches that, when applied to injuries, would increase the speed of healing,” said Dalton Tay, Assistant Professor from the School of Materials Science and Engineering.
The team aims to use the biomaterials-engineered MSCs secretome to treat chronic wounds and vascular diseases in humans and indicates that their hydrogel method could potentially be scaled up for mass production of healing factors in industry.
The venom factory
One type of tissue model used in drug discovery is organoids, which present the opportunity to study how cells of a certain organ may respond to disease and therapeutics.
…[factors in venom] affect systems as varied as the brain, neuromuscular junctions, blood coagulation and more. Many of them have potential bioprospecting applications for new drugs”
The study, published in Cell, detailed the development of functional Cape coral snake (Aspidelaps lubricus cowlesi) venom glands,4 demonstrating how researchers can use tissue engineering to increase the availability of a biological compound or secreted factor. In the anti-venom market, this kind of development could enable the mass production of venom for research without the need to keep or handle potentially lethal reptiles.
The typical process of venom ‘milking’ snakes can only produce a finite amount of venom at a time and the limited number of venomous reptile handlers and breeders results in limited development in anti-venoms, demonstrating the need for a method of production.
Senior study author Dr Hans Clevers of the Hubrecht Institute for Developmental Biology and Stem Cell Research at Utrecht University, Netherlands said: “More than 100,000 people die from snake bites every year, mostly in developing countries. Yet the methods for manufacturing antivenom haven’t changed since the 19th century.”
He also suggested that the research was valuable for development of other therapies as “every snake has dozens of different components in their venom…They affect systems as varied as the brain, neuromuscular junctions, blood coagulation and more. Many of them have potential bioprospecting applications for new drugs.”
Functioning organs
By themselves, organoids offer a powerful research tool but a new study has shown that greater value could be gained by modelling whole organ systems. Published in Nature, scientists reported how they grew a connected set of three organs: the liver, pancreas and biliary ducts.5
The researchers created induced pluripotent stem cells (iPSCs) from human skin cells and guided these cells through the process of forming two very early-stage “spheroids” of cells, vaguely representative of the foregut and the midgut in embryo development.
…this advance could reduce the need for animal models and sharply accelerate the development of precision medicine”
According to the researchers, while growing the spheroids was complex, the formation of the system was not; once they were mature, the spheroids were placed next to each other in a specially designed lab dish. The cells were suspended in a gel commonly used to support organoid growth, before being placed on top of a thin membrane that covered a carefully mixed batch of growth medium.
The lab team observed interactions between the organoid cells; converting into more specialised cells. The culture then sprouted branches leading to new groups of cells belonging to specific organs. Over a period of 70 days, these cells multiplied until the organoids began processing bile acids, as if they were digesting and filtering food.
“This was completely unexpected. We thought we would need to add ingredients or other factors to push this process,” revealed first author Dr Hiroyuki Koike at the Nippon Medical School in Japan.
According to the researchers, this advance could reduce the need for animal models and sharply accelerate the development of precision medicine. They also hope it may lead to transplantable tissues for regenerative therapies grown in labs.
Repairing a broken heart
While small sections of tissue such as organoids can survive, developing full-sized organs has so far been out of reach. A research team looking to overcome both this limitation and the shortage of organs for transplant have published a study in Stem Cell Reports showing their SWIFT 3D printing method can produce viable, life-sized, functional organs.6
Collaborators from the Wyss Institute for Biologically Inspired Engineering and John A. Paulson School of Engineering and Applied Sciences (SEAS), both US, pioneered a method of 3D printing vascular channels into living matrices composed of iPSC-derived organ building blocks (OBBs). The result is a fully vascularised mass of organ-specific tissue with high cell density and function.
Co-first author Dr Mark Skylar-Scott, Research Associate at the Wyss Institute, explained: “This is an entirely new paradigm for tissue fabrication… Rather than trying to 3D-print an entire organ’s worth of cells, SWIFT focuses on only printing the vessels necessary to support a living tissue construct that contains large quantities of OBBs, which may ultimately be used therapeutically to repair and replace human organs with lab-grown versions containing patients’ own cells.”
SWIFT involves a two-step process:
- Form iPSC-derived aggregates into a dense, living matrix of OBBs that contains about 200 million cells per millilitre
- Print a vascular network for oxygen and nutrients to support the tissue, by writing with, setting and then removing a strand of sacrificial gelatine ‘ink’.
The result is a network of channels embedded within the tissue that can be perfused with oxygenated media to nourish the cells, retaining their viability.
To assess whether the tissues displayed organ-specific functions, the team used SWIFT in a matrix of heart-derived cells and over the course of a week observed the cardiac OBBs fused together to form a more solid tissue, with contractions that became synchronised and over 20 times stronger than when they began.
Further research is underway to implant these organs into animal models and explore their host integration, with a view to establishing whether this technique could provide transplant organs comprising a patient’s own tissues.
Conclusion
Overall, the uses for stem cells are broad and expanding. Whether applied as a model of disease or drug action, a method for producing drugs or as possible transplant organs, stem cells have a lot to offer the field of drug discovery.
References:
- Deng Z, Wang W, Xu X, et al. Polymeric sheet actuators with programmable bioinstructivity [Internet]. 13 January 2020. PNAS. [cited 22 January 2020] Available at: https://www.pnas.org/content/early/2020/01/10/1910668117
- Ernst P, Yang W, et al. Enhancing Hematopoiesis from Murine Embryonic Stem Cells through MLL1-Induced Activation of a Rac/Rho/Integrin Signaling Axis [Internet]. 16 January 2020. Stem Cell Reports. [cited 22 January 2020] Available at: https://www.cell.com/…
- Yang H, Ji Cheam NM, et al. Materials Stiffness‐Dependent Redox Metabolic Reprogramming of Mesenchymal Stem Cells for Secretome‐Based Therapeutic Angiogenesis [Internet]. 18 September 2019. Advanced Healthcare Materials. [cited 22 January 2020] Available at: https://onlinelibrary.wiley.com/doi/abs/10.1002/adhm.201900929
- Clevers H, et al. Snake Venom Gland Organoids [Internet]. 23 January 2020. Cell. [cited 23 January 2020] Available at: https://www.cell.com/cell/fulltext/S0092-8674(19)31323-6
- Takebe T, Koike H, et al. Modelling human hepato-biliary-pancreatic organogenesis from the foregut–midgut boundary [Internet]. 25 September 2019. Nature. [cited 23 January 2020] Available at: https://www.nature.com/articles/s41586-019-1598-0
- Skylar-Scott MA, Lewis JA, et al. Biomanufacturing of organ-specific tissues with high cellular density and embedded vascular channels [Internet]. 6 September 2019. Science Advances. [cited 23 January 2020] Available at: https://advances.sciencemag.org/content/5/9/eaaw2459
Related topics
Antivenoms, Bioengineering, Biopharmaceuticals, Biotherapeutics, Cell Regeneration, Disease Research, Drug Targets, Induced Pluripotent Stem Cells (iPSCs), Organoids, Protein, Protein Expression, Research & Development, Stem Cells, Therapeutics
Related conditions
bone cancer, bone fractures, Diabetes, Leukaemia, Osteoporosis, vascular disease
Related organisations
Helmholtz-Zentrum Geesthacht, John A. Paulson School of Engineering and Applied Sciences (SEAS), Nanyang Technological University, Nippon Medical School - Japan, Singapore (NTU Singapore), University of Colorado (CU) Cancer Center, Utrecht University, Wyss Institute for Biologically Inspired Engineering